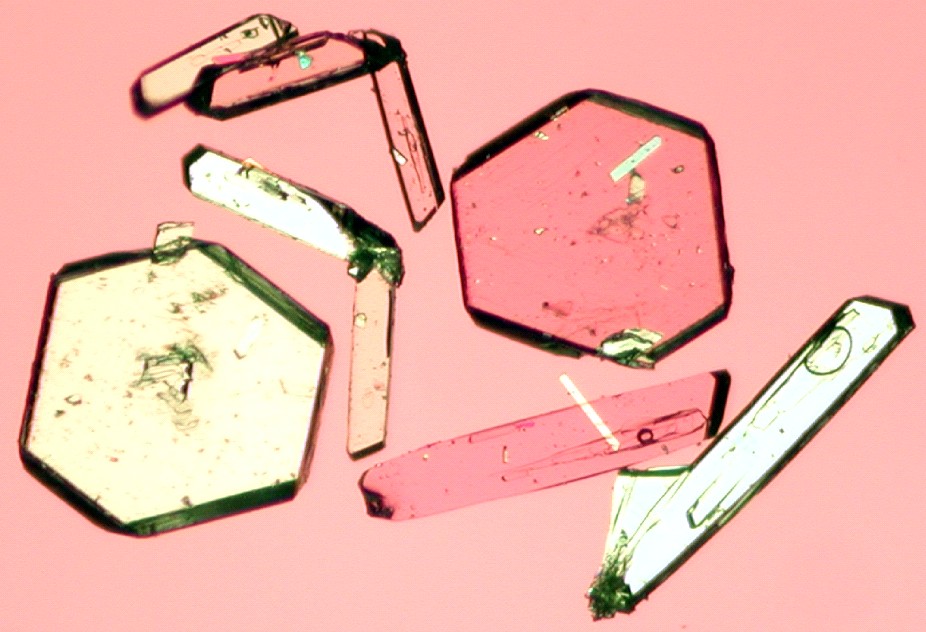
DRUG POLYMORPHISM
Polymorphism which is common in active pharmaceutical ingredients (APIs), is the ability of a substance crystallization into more than two crystalline forms.
-
Polymorphism in Pharmaceutical Solids (Drugs and the pharmaceutical sciences), 2nd ed.; Brittain, H. G., Ed.; Informa Healthcare: New York, 2009.
-
Hao, H.; Barrett, M.; Hu, Y.; Su, W.; Ferguson, S.; Wood, B.The Use of in Situ Tools To Monitor the Enantiotropic Transformation of p-Aminobenzoic Acid Polymorphs Org. Process Res. Dev. 2012, 16 ( 1) 35–41, DOI: 10.1021/op200141z
For the different arrangements in crystal lattices, different polymorphs of APIs have various stability, solubility, and bioavailability.
-
Butterhof, C.; Martin, T.; Ectors, P.; Zahn, D.; Niemietz, P.; Senker, J.Thermoanalytical Evidence of Metastable Molecular Defects in Form I of Benzamide Cryst. Growth Des. 2012, 12 ( 11) 5365– 5372, DOI: 10.1021/cg3009706
-
Delaney, S. P.; Pan, D.; Galella, M.; Yin, S. X.; Korter, T. M.Understanding the Origins of Conformational Disorder in the Crystalline Polymorphs of Irbesartan Cryst. Growth Des. 2012, 12 ( 10) 5017– 5024, DOI: 10.1021/cg300977e
-
Su, W.; Hao, H.; Glennon, B.; Barrett, M.Spontaneous Polymorphic Nucleation ofd-Mannitol in Aqueous Solution Monitored with Raman Spectroscopy and FBRM Cryst. Growth Des. 2013, 13 ( 12) 5179– 5187,DOI: 10.1021/cg400304c
Generally, the thermodynamic stable crystal form would be selected for formulation. However, the thermodynamic metastable crystal with better solubility and bioavailability, would also be adopted for the pharmaceutical preparation.
Process analytical technology (PAT) is an efficient tool to monitor the polymorphic transformation process. Brian O’Sullivan and co-workerscombined the focused beam reflectance measurement (FBRM) probe and the react infrared (react IR) probe to monitor the polymorphic transformation process of d-mannitol.
-
Herman, C.; Haut, B.; Douieb, S.; Larcy, A.; Vermylen, V.; Leyssens, T.Use of in Situ Raman, FBRM, and ATR-FTIR Probes for the Understanding of the Solvent-Mediated Polymorphic Transformation of II–I Etiracetam in Methanol Org. Process Res. Dev. 2012, 16 ( 1) 49– 56, DOI: 10.1021/op200168g
-
Jia, C.-Y.; Yin, Q.-X.; Zhang, M.-J.; Wang, J.-K.; Shen, Z.-H.Polymorphic Transformation of Pravastatin Sodium Monitored Using Combined Online FBRM and PVM Org. Process Res. Dev. 2008, 12 ( 6) 1223–1228, DOI: 10.1021/op8001257
-
Takeguchi, K.; Obitsu, K.; Hirasawa, S.; Orii, R.; Ieda, S.; Okada, M.Effect of Temperature and Solvent of Solvent-Mediated Polymorph Transformation on ASP3026 Polymorphs and Scale-up Org. Process Res. Dev. 2016, 20 ( 5) 970– 976, DOI: 10.1021/acs.oprd.6b00068
-
Howard, K. S.; Nagy, Z. K.; Saha, B.; Robertson, A. L.; Steele, G.; Martin, D.A Process Analytical Technology Based Investigation of the Polymorphic Transformations during the Antisolvent Crystallization of Sodium Benzoate from IPA/Water Mixture Cryst. Growth Des. 2009, 9 ( 9) 3964– 3975, DOI: 10.1021/cg900108e
Flexible carbon chains with a polar or heterocyclic endgroup often occur in modern drugs and give conformational flexibility but also additional hydrogen bonding possibilities (with the headgroup). However, it is fair to say that several older drugs (e.g., chlorpromazine) were also of this type.
Of course, it could be that chemists are much more aware of polymorphism and solvation issues and thus are looking out for potential problems as well as getting involved with projects at an earlier stage. Thus, preliminary screening for polymorphs can, in some companies, occur before candidate selection. Also, the tools for screening for crystal forms have improved, with more automation meaning that the number of experiments carried out is increased, thus ensuring that, as far as is possible, all forms are detected in the screening process (though this will depend on a number of factors including the quality, particularly the number and level of key impurities, of the sample of API used in the testing).
It is clear that an understanding of crystallisation and polymorphism must be part of the skills of the organic development chemist (as well as the chemical engineer who is often taught these topics at undergraduate level) and that specialist training is required to bring organic chemists up-to-speed with current advances and knowledge. Development chemists love to tinker with the chemistry but must be made aware of the possibility of changing the crystal form of the product (API or intermediate) when they do. Whereas obtaining a new, more stable crystalline form of an intermediate is usually good news (since the lower solubility may allow a yield increase in the crystallisation stage), a more stable form of an API may be a mixed blessing, depending on how far the project is in development.

The crucial need for exploring solid-state properties is commonly recognized in the pharmaceutical industry.
-
Henck, J.-O.; Byrn, S. R. Drug Discovery Today 2007, 12, 189– 199
-
Byrn, S. R.; Zografi, G.; Chen, X. J. Pharm. Sci. 2010, 99, 3665– 3675
-
Palucki, M.; Higgins, J. D.; Kwong, E.; Templeton, A. C. J. Med. Chem. 2010, 53, 5897– 5905
-
Gibson, M.Early Drug Development: Product Design. In Pharmaceutical Preformulation and Formulation;Gibson, M., Ed.; Interfarm/CRC: London, New York, WA, 2004.
Ideally, the in vivo efficiency, pharmacokinetics, and toxicology studies should be performed with the drug candidates that exhibit optimal solid-state properties. As indicated by Huang and Tong. the most appropriate time for finding a suitable solid form is during the lead identification (LI) and lead optimization (LO) phases of the drug discovery process. At the later stages, the developability assessment criteria for new drug candidates are applied to identify the potential challenges and even project “stoppers” of the drug development.
-
Huang, L.-F.; Tong, W.-Q. Adv. Drug Delivery Rev. 2004, 56, 321– 334
-
Bernstein, J. Cryst. Growth Des. 2011, 11, 632– 650
-
Balbach, S.; Korn, C. Int. J. Pharm. 2004, 275, 1– 12
These criteria are typically set to solubility and dissolution, hygroscopicity, stability, and synthesis process scalability of the chosen solid-state form. Estimation of scalability of the synthesis process and physical stability includes evaluation of the tendency of a new chemical entity to crystallize in different crystal forms, investigation of thermodynamic relationship between polymorphs and solvates as well as relative stability of possible solid forms at ambient conditions.
A key element of the strategy to reduce the time required for preclinical development is to link solid-form discovery with formulation, formulation design, and manufacturing.
(1) According to Byrn et al., the most efficient way to speed up new drug development is by means of accelerating the process of ‘proof-of-concept’ (POC). Specifically, this acceleration can be achieved by performing the best solid-form screenings as soon as the candidate has been selected. Knowledge about the
polymorphism and solvatomorphism tendency and solid-state properties of the new candidate is of great importance for screening facilitation.
The polymorphism and solvatomorphism tendency has been the subject of numerous investigations. It is traditionally studied through crystallization from various solvents using diverse temperature profiles and concentrations, as well as by applying an additional thermal, pressure, or mechanical treatment. Due to a limited amount of substance available at the early discovery phases, most screening methodologies were adapted for using small-scale crystallization volumes and miniaturized analysis techniques. As a result, in all these approaches designed for the early discovery phase, the crystallization conditions differ significantly from those used in ordinary organic synthesis processes. First, the crystallization experiments are performed in very small (microliter scale) volumes, and second, they typically do not involve any real synthesis. These small-volume approaches have a tendency to produce metastable and amorphous forms, as well as the mixtures of them. As a result of which the most stable form can remain undiscovered. Consequently, there is a practical need for development cost-effective polymorphism and solvatomorphism tendency evaluation approaches directed on discovery of the stable solid form at ambient conditions.
In this context, we have chosen a rational approach for the fast initial
polymorphism and solvatomorphism tendency evaluation tendency evaluation at the very beginning of the discovery process. The systematic examination of solid state properties of sequentially synthesized batches is merged with synthesis process optimization thus enabling assessment of
polymorphism and solvatomorphism tendency on the real process scale. This approach is schematically presented in Figure
1. Briefly, the physical characterization of each fresh batch of a new substance is performed by means of standard methods, X-ray powder diffraction (XRPD), differential scanning calorimetry (DSC), and thermal gravimetric analysis (TGA). Simultaneously, in order to evaluate the physical stability at high relative humidity, every single batch is analyzed by the gravimetric vapor sorption (GVS) method combined with XRPD and TGA analysis performed before and after GVS. The final crystallization step of the synthesis is adjusted until a stable solid form can be reproducibly crystallized.
-
Byrn, S. R.; Pfeiffer, R. R.; Stowell, J. G. Solid-State Chemistry of Drugs; SSCI Inc: West Lafayette, 1999.
-
Vippagunta, S. R.; Brittain, H. G.; Grant, D. J. W. Adv. Drug Delivery Rev. 2001, 48, 3– 26
-
Almarsson, O.; Hickey, M. B.; Peterson, M. L.; Morissette, S. L.; Soukasene, S.; McNulty, C.; Tawa, M.;MacPhee, J. M.; Remenar, J. F. Cryst. Growth Des. 2003, 927– 933
-
Brittain, H. G. J. Pharm. Sci. 2007, 96, 705– 728
-
Hilfiker, R. Polymorphism in the Pharmaceutical Industry; Wiley-VCH, Verlag GmbH: Weinheim, 2006.
-
Descamps, M.; Willart, J. F.; Dudognon, E.; Caron, V. J. Pharm. Sci. 2007, 96, 1398– 1407
-
Nangia, A. Acc. Chem. Res. 2008, 41, 595– 604
-
Aaltonen, J.; Alleso, M.; Mirza, S.; Koradia, V.; Gordon, K. C.; Rantanen, J. Eur. J. Pharm. Biopharm. 2009,71, 23– 37
-
Brittain, H. G. Polymorphism in Pharmaceutical Solids; Marcel Dekker Inc.: New York, 2009.
-
Gardner, C. R.; Almarsson, O.; Chen, H.; Morissette, S.; Peterson, M.; Zhang, Z.; Wang, S.; Lemmo, A.;Gonzalez-Zugasti, J.; Monagle, J.; Marchionna, J.; Ellis, S.; McNulty, C.; Johnson, A.; Levinson, D.; Cima,M. Comput. Chem. Eng. 2004, 28, 943– 953
-
Morissette, S. L.; Almarsson, O.; Peterson, M. L.; Remenar, J. F.; Read, M. J.; Lemmo, A. V.; Ellis, S.; Cima,M. J.; Gardner, C. R. Adv. Drug Delivery Rev. 2004, 56, 275– 300
-
Aaltonen, J.; Strachan, C. J.; Poellaenen, K.; Yliruusi, J.; Rantanen, J. J. Pharm. Biomed. Anal. 2007, 44,477– 483
New Drugs
The US Food and Drug Administration (FDA) require submitters of an Investigational New Drug Application (INDA – new drug) to submit polymorph information.
New Generic Drugs
The US FDA requires submitters of an Abbreviated New Drug Application (ANDA – new generic) that they must include a polymorphism study in order to demonstrate equivalence.
In addition, the International Conference on Harmonisation of Technical Requirements of Pharmaceuticals for Human Use (ICH harmonised Tripartite Guideline) ICHQ6A states that the following is required: Evidence that polymorphism is or is not exhibited by a new drug substance. If polymorphism is exhibited, whether the different polymorphic forms can affect performance of the drug product, and what the potential for change is and how it might be controlled.
Drug Development
“Polymorphic forms of a drug substance can have different chemical and physical properties, including melting point, chemical reactivity, apparent solubility, dissolution rate, optical and mechanical properties, vapour pressure, and density. These properties can have a direct effect on the ability to process and/or manufacture the drug substance and the drug product, as well as on drug product stability, dissolution, and bioavailability. Thus, polymorphism can affect the quality, safety, and efficacy of the drug product.” [FDA guidelines]. In simple visual terms, the following scheme shows how to envisage polymorphism. The Drug Substance is shown as a rectangle which can pack together in a crystal in different arrangements. Each separate arrangement is a different polymorphic form. It is believed that 50-80% of all Drug Substances exist in at least 2 polymorphic forms
Polymorph A
Polymorph B
Each stage in drug development gets increasingly more complex. After finding a potential hit in a screen, the early drug development phase of ‘hit to lead’ follows. This ‘lead’ drug is then optimised and prepared for pre-clinical evaluation. Drug development during the pre-clinical phase is designed to determine a drug’s safety profile and prepare the drug for use in clinical trials. During drug development, an initial scouting polymorph screen is designed to find a stable non-solvated form with good properties. Phase 1 clinical trials are the first time during pharmaceutical development that the drug is used in humans to test safety and tolerability. Larger Clinical Phase 2 studies assess how well the drug works. This is followed by Clinical Phase 3 trials, the most expensive of all, to assess drug effectiveness. Phase 3 drug development work includes a comprehensive polymorph screen find as many forms as possible in order to exhaustively cover the Intellectual Property space. Continuous monitoring of the polymorphic form is needed throughout the whole drug development process in order to ensure consistent manufacture of the specified polymorph
Analytical techniques are powerful tools employed during drug development: X-ray powder diffraction is used to provide unequivocal proof of polymorphism. Other methods, including Thermal Analysis; Differential Scanning Calorimetry (DSC), Thermal Gravimetric Analysis (TGA), Hot-Stage Microscopy (HSM) and Raman spectroscopy are useful to further characterise polymorphic forms.
The consequences of Abbott Laboratories’ antiretroviral drug Ritonavir, used to treat HIV infection and AIDS and problems with polymorphism has yet to be universally understood. Avoiding action has not yet widely been taken on polymorphism by the global pharmaceutical industry, so it is worth repeating the story.
In 1996 Abbott launched on the market an effective protease inhibitor Norvir® that had cost the company in excess of $200 million to develop. The drug was formulated as an encapsulated ethanol/water solution. In the summer of 1998, supplies of the drug were interrupted by the appearance of a new crystal form (polymorphism) at a plant in the USA and then later at a plant in Italy. This new, more thermodynamically stable polymorphic form had very different physical properties than the earlier material and Abbott was forced to withdraw the drug from sale. The new form failed dissolution tests and precipitated out within the capsules. The company lost an estimated $250 million in sales as well as hundreds of millions of dollars trying to recover the original polymorph while the product was off the market. No doubt many AIDS sufferers were not helped by the product’s absence. What appeared to have happened was that a degradation product obtained during manufacturing had initiated the appearance of a second crystalline form, a second polymorph.
So what is polymorphism? It is simply a different arrangement a molecule might adopt in a crystal. Most drug molecules are crystalline. That is, the molecules pack together in a particular regular way. Some molecules, perhaps most, are able to pack together in more than one way and thus give rise to different polymorphs. A pair of polymorphs might have very different physical properties. Over 50% of all Active Pharmaceutical Ingredients (APIs) exist in at least 2 polymorphic forms.
During drug development, an initial scouting polymorphism screen is designed to find a stable non-solvated form with good properties. A later comprehensive polymorphism screen is to find as many forms as possible in order to exhaustively cover the Intellectual Property space. Continuous monitoring is needed throughout development in order to ensure continued control of polymorphism.
What can cause polymorphism changes? Crystallisation from different solvents may give rise to different crystal forms or solvates. Extremes of humidity or heat are among the more obvious factors affecting polymorphism. Changes in polymorphism can also be induced as a consequence of several common stages of API processing such as granulation, melting, spray drying, compression and milling that are required to produce the final dosage form.
X-Ray Powder diffraction and Raman spectroscopy are the two workhorse methods used to evaluate the presence and amount of different polymorphic forms found during screening. Differential Scanning Calorimetry (DSC), Differential Thermal Analysis (DTA), Dynamic Vapour Sorption (DVS) and hot stage microscopy are additional specialised techniques used to characterise different polymorphic forms.
All is not doom and gloom as the polymorphism properties of a chemical substance are patentable. A new polymorphic form therefore may be used to extend the patent life of a drug. A counter to this is that a new polymorphic form with advantageous properties may be able to supersede an existing drug and effectively ‘bust’ the original patent.
It’s not surprising to learn that manufacturers & developers of generic drugs actively pursue new polymorphic forms of patented drug substances. This can be a highly litigious area.
National regulatory authorities require that all companies register the precise polymorph of any new drug. Moreover, manufacturers need to demonstrate that each polymorph is stable and can be reliably reproduced
Many small pharmaceutical companies do not intend taking their drug candidates all the way to commercialisation themselves but to seek to license at an earlier stage. This arises because of the considerable increase in costs as a drug moves through the clinic. If a polymorph screen is also included then the package is certainly stronger and the licensor can expect a further premium.
During pre-clinical development the quantity of an API that is available for studies is usually very low. Screening a wide variety of solvents and conditions needs to be conducted for that reason on a very small scale. Systems that can handle multiple experiments at the milligram scale are best. At some labs automated systems can handle up to 96 well plate formats and conduct experiments at 0.5-2mg scale. Thus the total amount of API used for an initial screen can be a modest 50-200mg.
Typically, a salt selection project will precede a polymorphism study: once a salt is found that has the most promising properties, it will be further developed, characterised and might be the subject of a further polymorphism screen during the normal course of pre-clinical development.
Polymorphism, in addition to complicating drug development also aids drug efficacy and can ensure that any hard earned work is justifiably rewarded.
In the current economic climate more and more pharmaceutical companies are recognising the need to optimise the physical form of their new candidate drugs and try to ensure that they maximise their intellectual property (IP) at the same time. The polymorphic form of a solid substance, including most drugs, is patentable and it is theoretically possible to find a new polymorphic form of an existing drug and patent it. If the new polymorphic form has advantageous properties over the existing product on the market, it can, in principle supersede the earlier product. Moreover, if a new polymorphic form suddenly appears (it does happen), it may have properties that are inconsistent with its current formulation. One polymorphic form may be more biologically available than its predecessor or it may be less biologically available. Either way, a lot of money may have been wasted developing a particular polymorph. Recent examples show that it can be very costly if the physical form is not controlled but the pharmaceutical industry has not yet fully embraced this aspect of drug development.
So what is polymorphism? It is simply a different arrangement a molecule might adopt in a crystal. Most drug molecules are crystalline. That is, the molecules pack together in a particular 3 dimensional way. Some molecules, perhaps most, are able to pack together in more than one way and give rise to different polymorphs. A pair of polymorphs might have very different physical properties. It is the skill of the chemist, employed during the process development of the drug, that ensures the desired polymorph is consistently made. Another type of chemist, employed to research the possible variations on polymorphism, could also be extremely effective in ensuring that all bases are covered from an IP perspective.
These days, we all need to keep an eye on the bottom line. Perhaps if your company was to cast an eye over the other lines as well and make use of specialists inpolymorphism to do this research for you, you wouldn’t have to worry so much about your future prospects!
New Polymorphic Form
The vast majority of countries do not present a specific mention in the Law for polymorphic form patent application, but still many patents are granted. The polymorphic forms are expressly regulated in India, as long as patentability requirements are met and efficiency is increased.
Both second medical use and new polymorphic forms are currently patentable according to Law, evaluating the novelty and inventive activity in Brazil; however, the guidelines were not clear. A guideline proposal for the evaluation of patent application for new polymorphic forms is being prepared.
Guideline Proposal for the Evaluation of new polymorphic forms
– Definitions were established for: polymorphism, crystalline and amorphous forms, medicine, pharmaceutical composition, pharmaceutical formulation, adjuvant, excipient and monocrystal.
– It is indispensable that the descriptive report has the necessary characteristics to the complete description of new polymorphic forms, according to technologies inherent to its perfect characterization, such as the following examples, or validated alternative techniques that best identify them:
a) Single-crystal X-ray diffraction (monocrystal XRD);
b) X-Ray Powder Diffraction Method; (XRD Powder Method);
c) Solid-state carbon-13 nuclear magnetic resonance spectroscopy (NMR-13C)
d) Infrared Spectroscopy;
e) Raman Spectroscopy;
f) Electronic Microscopy (hot stage);
g) Thermal Analysis: Differential Scanning Calorimetry (DSC), Thermogravimetric Analysis (ATG) and Differential Thermal Analysis (DTA).
– In case of a monocrystal of the chemical substance, the XRD technique of single crystal is sufficient for the prefect characterization of the crystalline structure.
In case of absence of monocrystal of the chemical substance, the X-Ray Powder Diffraction Method technique with indexing must be used, that is, the Miller index of the interplanar distances, the Bragg angles and the relative intensities of at least 20 reflections must be presented, in addition to the unitary cell parameters.
– If the indexing is not presented, the presentation of the other described parameters is necessary, and also the complementation of the X-Ray Powder Diffraction Method with other analysis methods (minimum of 2), in addition to the proof of sample purity.
The analysis technique used for the determination of the chemical substanceand its purity level pharmaceutically acceptable must be informed, since sample impurities may cause interferences in the quality of the characterization analysis results of the crystalline structure.
The inventive activity of a polymorphic form shall be checked by verification of an effect solving a problem of the prior art that is sufficiently differentiating, for instance: stability increase, apparent solubility and processability.
Pharmaceutical Composition of the Polymorphic Form
A composition is a grouping of ingredients for a certain purpose. The composition presents a novelty when one of the constituents is a crystalline form different from the one disclosed in the prior art.
The inventive activity to be presented by the composition containing the new polymorphic form, there must be an evaluation of the differentiated effect authentically solving a technical problem, based on its giving special attention to comparative specific parameters in relation to the prior art, the proof of its physical and chemical stability for its industrial use.
Process for Obtaining the Polymorphic Form
The process must be new, inventive and clearly described in order to allow the reproducibility by a person skilled in the art.
Parameters as exemplified below, or alternative parameters best describing such process, must disclose the following:
a) concentrations of the different solutions used, which are critical to the process;
b) solvents;
c) cooling rate;
d) time;
e) temperature of the different stages of the process;
f) torque or addition of seeds of the desired crystalline form. The seed preparation process must also be clearly and sufficiently described in the application when the crystallization process is developed with seeding.
The same criteria are applied to the pharmaceutical and medication formulation, repressing it.
Process for Obtaining the Pharmaceutical Composition
The process for obtaining a pharmaceutical composition containing a new polymorphic form is new. The inventive activity shall be evaluated whenever it is not evidently originating from the prior art (for the person skilled in the art).
As new polymorphic forms may disclose different physical and mechanic properties, including particle size, density, hardness, outflow and compactness, they may have sufficiently differentiated characteristics solving a problem of the prior art, which may bring an inventive activity requisite to the obtaining process of the pharmaceutical composition.
The same criteria apply to the process for obtaining the pharmaceutical and medication formulation.
CASE STUDY
Polymorphism
Acetazolamide (ACZ)
Acetazolamide is used mainly in treatment of glaucoma. It is also used as an antiepileptic and anti diuretic agent. It is formulated as tablets, capsules (for extended release) and injection. It is commercially available as Diamox.

Figure 3: The structure of Acetazolamide (Jenniffer I. Arenas-Garcı´a et al.2010)
The above fig 3 shows the structure of acetazolamide. It has two known polymorphic forms (Form A and B). Form A being the more stable form than form B. Acetazolamide has low solubility and permeability. Hence efforts are being made to form and identify new co- crystals forms of the drug (Jenniffer I. Arenas-Garcı´a et al.2010). Co crystals were made by using four different techniques. Co crystals were prepared by using Solvent drop grinding experiments. Out of 20 Co crystal former used 4-Hydroxybenzoic Acid and Nicotinamide were found to form stable co crystals with acetazolamide. Co crystals formed were characterized using XRPD, IR spectroscopy, DSC and TGA techniques.

Figure 4: Crystals of acetazolamide (ACZ) and the corresponding co-crystal with 4-hydroxybenzoic acid (ACZ-4HBA) can be distinguished by their different shapes (Jenniffer I. Arenas-Garcı´a et al.2010).
Co crystal phase stability was carried out to check whether the co crystals formed convert to the parent compound ie.ACZ. The co crystals were exposed to water at various physiological pH values. The co crystal formed by ACZ with nicotinamide transformed to ACZ and this was time and pH dependent while co crystal with 4 -hydroxybenzoic acid didn’t transform into ACZ.

Figure 5: Molecular conformations of ACZ in the crystal structures of ACZ form A,9a,d AC form B,9c ACZ-4HBA, and ACZNA- H2O. N4-S2-C1-S1 torsion angles are indicated (Jenniffer I. Arenas-Garcı´a et al.2010).
Figure 5 gives the molecular conformation of the available polymorphs of ACZ and the two new co crystals formed. These structural details demonstrate that ACZ possesses two potential sites for the formation of doublebridged homo- and heterosynthons, the carboxamidine (C(N)NH) group on the thiadiazole acetamide fragment and the sulfonamide group(Jenniffer I. Arenas-Garcı´a et al.2010).
Thus the co crystals formed were stable enough at ambient temperature. However this study fails to compare the solubility and permeability of co crystals with ACZ.
Paracetamol

Figure 6 : The Structure of paracetamol (Zimmermann B et al. 2010)
Paracetamol is used as an antipyretic and analgesic. Commercially it is available as tablets, capsules, solutions, suspensions etc. Paracetamol has three known polymorphic form viz. Monoclinic (Form I), Orthorhombic (Form II) and a third instable form III. Efforts were made in characterising form III with use of rapid heating DSC and HPMC (crystal growth modifier) (S. Gaisford. et.al. 2010). Thermal analysis of the polymorphs was carried out using FT-IR spectroscopies (Zimmermann B et al. 2010). Form II is the commercially available form of paracetamol. However form I has a stiffer arrangements in the crystal lattice compared to the orthorhombic metastable form II. Hence form I shows poor compression ability. (Etienne Joiris et.al.1998) Although form II shows better compression ability compared to form I but still form I is preferred as API. This is due to then stability issues related with form II. The stability of the form II varies with alteration in temperature and humidity. Hence, the stability issue prompts the manufacturer to liken form I. Efforts should be made to make form II more stable under ambient temperature and humidity.
Itraconazole

Figure 7: The Structure of Itraconazole (Julius F. Remenar et al.2003)
Itraconazole (Fig 7) is an antimicrobial agent. It is commercially used for treatment of onychomychosis. The main disadvantage for this drug is its solubility. It is a poorly soluble drug. Hence attempts were made to form a co crystal of the drug which will increase the solubility of the drug. Amorphous state of the drug has a greater solubility than the crystalline form but the amorphous form is less stable and degrades quickly in presence of humidity and temperature. Hence in one such attempt a co crystal of itraconazole was prepared using 1,4-dicarboxylic acids (Julius F. Remenar et al.2003). A trimeric co crystal was prepared with itraconazole atoms at the two ends of the dicarboxylic acid (Succinic acid).

Figure 8:Dissolution profile of itraconazole
From the fig 8 we can conclude that the co crystal with malic acid matches the solubility that of the amorphous itraconazole (Julius F. Remenar et al.2003). Thus this study suggests that it is possible to have a form which matches the bioavailability of the amorphous form but has the physical and chemical stability of the crystalline form.
Fluoxetine

Figure 9:Co crystal of Fluoxetine – Succinic acid (Scott L. Childs et al. 2004)
Fluoxetine is a selective serotonin reuptake inhibitor. It is indicated for acute and maintenance treatment of Major Depressive Disorder (MDD), Obsessive Compulsive Disorder (OCD), and Panic Disorder. (Drugs@FDA). It is commercially marketed as tablets, capsules (for sustained release) and solution (discontinued). Crystalline form of the drug shows poor solubility. Hence efforts were made to develop a co crystal which will have increased solubility compared to the crystalline form. Co crystals were prepared using 1,4-dicarboxylic acids (Scott L. Childs et al. 2004). The above figure 9 shows the co crystal formation between fluoxetine and succinic acid. Co crystallization takes place due to hydrogen bond interactions. The formation of co crystal was confirmed with single crystal structure X-Ray diffraction and IR spectroscopy.

Figure 10: Dissolution profile of the co crystals (Scott L. Childs et al. 2004)
From the fig 10 we can see that distinctive dissolution profiles can be achieved through co crystallization. Hence these forms of API’s can be used to make alternate formulations.
Amorphous Forms
Celecoxib
CELECOXIB is a nonsteroidal anti-inflammatory drug indicated for Rheumatic arthritis. It acts as a COX2 inhibitor. It is commercially marketed as CELEBREX and is supplied in the form of capsules.

Figure 11: The structure of Celecoxib (Patent No. U.S. 6.964,978, 2005)
The crystalline form of the drug is marketed. However it was found that the higher bioavailability was shown by the amorphous state. The downfall of the amorphous state was its stability. This was due to the structural relaxation which causes devitrification of the celecoxib if stored at room temperature (K. Grzybowska et al. 2010). This was enhanced by mixing it with polymers like PVP, PVP-Meglumine which helped in stabilising the amorphous system (Piyush Gupta et al. 2004, Piyush Gupta et al. 2005). A new solid state form was developed by Pharmacia Corporation and patented (Patent No. U.S. 6.964,978, 2005). The polymer used form a composite with the amorphous form and prevents its conversion to the crystalline form. Crystalline form of the Celecoxib is less pharmaceutically active. The amorphous lactose was prepared from the crystalline one by spray drying techniques with isopropanol. Studies were conducted to check the stability and solubility of the drug- polymer amorphous system (Piyush Gupta et al. 2004). The measurement of the crystalline content, glass transition temperatures and enthalpy relaxation were characterised using DSC. Dissolution and solubility studies were carried out to ceck the release mechanisms. Hence by these techniques the new form of Celecoxib was discovered and an improved formulation was developed.
Simvastatin

Figure 12: The structure of Simvastatin (Kirsten A. Graeser et al. 2008)
Simvastatin is a cholesterol reducing agent belonging to the statin family. It belongs to BCS class II (low solubility but high permeability). It has two known polymorphs, however the second polymorph requires extreme conditions to form and form I is the most known stable form available. Simvastatin is suitable candidate for amorphous form. In one such study the physicochemical properties of two differently prepared amorphous forms were prepared and characterized (Kirsten A. Graeser et al. 2008). The two methods were cryo-milling and quench cooling. In quench cooling the compound was melted in oven and then quench cooled by putting liquid nitrogen on the plate carrying the compound. In cryo milling the compound was ball-milled and the mill was surrounded liquid nitrogen. The amorphous material obtained by two different methods were further characterized by using FT-IR, DSC, High Speed DSC, TGA, XRPD, PLS and SEM.

Figure 13: SEM images of simvastatin (a) crystalline, (b) cryo-milled, (c) 4 °C milled, (d) RT milled, (e) recrystallized cryo-milled, and (f) quench cooled.( Kirsten A. Graeser et al. 2008)
Figure 13 depict the difference in the crystal structre of the amorphous material prepared by different techniques. Accelerated Stability studies were carried out on the amorphous material prepared by two techniques. The cryo- milled amorphous product showed less stability compared to quench cooled product. The logic behind making amorphous form is that it shows more solubility than the crystalline form. Amorphous form has more bioavailability compared to the crystalline form. The main disadvantage of this form is that it has excess of thermodynamic properties hence it tends to recrystallize into its crystalline form. If we are able to keep the amorphous form stable enough till the expiry of the medicine then we can achieve a novel and alternative formulation.
Polymorph Screening for Identification of Relevant Crystalline Forms
Gauging the adequate level and type of screening is the challenge when identifying relevant crystalline forms.
Solid-dosage drugs remain the preferred dosage form due to ease of administration and typically lower manufacturing costs compared to parenteral and other dosage forms. Many of the properties of a chemical relate directly to its physical form, and solid compounds can adopt numerous different crystalline forms (e.g., polymorphs, solvates, and hydrates) and non-crystalline (e.g., mesophases or amorphous) forms. Extensive screening must be completed to identify the different potential stable and meta-stable forms that an API may adopt during manufacturing of the drug substance and drug product, packaging, storage, and within the body. Potentially stable salts and cocrystals of the API may also be evaluated. In addition to the skills and knowledge required to conduct and evaluate the large quantities of structure data generated during polymorph screening projects, the ability to design appropriate screening studies has a direct impact on their success.
Why polymorph screening
Toxicology, efficacy, and stability are important criteria when selecting an appropriate candidate for the development of a solid-dosage drug. “These properties can vary depending on the physical structure of the API. It is necessary to understand the physical properties of each potential solid form and the relationship between these different forms in order to identify the candidates with the greatest likelihood of success,” says Brett Cowans, director of materials science for SSCI (Solid State Chemical Information), a division of AMRI.
The chances of selecting the best candidate improve considerably the earlier these factors are known. “The challenge for companies is to balance the cost of development and risk of selecting the wrong solid form. Ideally, this balance will include understanding the true physical properties of the selected candidate compound, including polymorphism, and how these properties will affect the API manufacturing and drug product efficacy and stability for each form, as well as the intellectual property position for the formulated drug,” Cowans observes.
Many forms to identify
From a technical point of view, the main challenge of polymorph screening is to identify all crystalline forms relevant and potentially crucial to development. Solid forms may, for example, be observed in the presence or absence of certain impurities in the final isolation step of the API, upon storage of its intended dosage form, or in vivo when administered orally, according to Patricia Andres, director of particle engineering for SSCI. “The screening of such forms typically requires a rational design of experiments often not amenable to high-throughput screening, and the unambiguous identification of new phases necessitates the preparation of pure phases in sufficient quantities for characterization,” she notes.
Andres cautions, however, that while thorough and targeted screening will considerably reduce the chances that a new critical form is discovered later in development, it is not possible to know without a doubt that all relevant forms have been found. She cites ritonavir as a classic example of a crystalline form not discovered during development. The product was developed using a form designated as Form I, which was later found to be metastable with respect to a new form exhibiting considerably lower solubility and rendering the initially developed drug product bio-unavailable.
Achieving the right balance
“The ability to gauge the level and type of screening that is adequate based on considerations of development phase, dosage form, physicochemical and biological properties of the compound, and regulatory requirements is paramount,” Andres asserts. Risks associated with the discovery of a new crystalline form at a later phase of development must be weighed against the possibility of the compound failing in development. This risk assessment requires both experience of solid-state issues that can affect the performance of the drug substance and drug product and intimate knowledge of the drug substance and drug-product processes.
Another practical challenge in polymorph screening, according to Marco Gil, a general manager with Hovione, is to identify processes for isolation of different forms that can be scaled in a robust manner to yield the selected polymorphs (or cocrystals).
Fit-for-purpose approach
Polymorph screening is a complicated process that involves many individual experiments and significant data analysis. Significant resources have been invested in efforts to accelerate the process. The dream of displacing rational experimental design in pharmaceutical form screening (e.g., salts, cocrystals, and polymorphs) with high-throughput screening was pursued for about a decade by mid-sized and large pharmaceutical companies, as well as material science contract research organizations (CRO), according to Jon Selbo, director of preformulation with SSCI. “Significant internal resources were dedicated to developing home-built screening instruments and/or purchasing equipment and software to implement this new strategy,” he says.
The justification for high-throughput screening was that significant improvement in identification of otherwise unknown forms could be more easily obtained by conducting large arrays of experiments in parallel. In most cases, however, this strategy failed for both identification of new forms and desired forms for development. “In general, crystallization quality was poor, there was a lack of experimental diversity (regardless of significant increases in solvents used in crystallization attempts), the analytical results were inadequate, and analyzing the large quantities of poor quality data generated in the experiments often proved difficult,” Selbo observes. In addition, polymorphs identified using high-throughput screening must be considered as general lead compounds and must be thoroughly assessed, including evaluation of their pharmacokinetics, solubility, and other properties, according to Gil.
The driver for large-scale screening in early development has, for the most part, also disappeared with the new molecular entities failing more often today due to efficacy and toxicity issues rather than biopharmaceutics properties. “Only a few larger pharmaceutical companies and small CROs continue to employ high-throughput screening as a first pass strategy,” says Selbo.
What is important, according to Cowans, is how the results of high-throughput screening can contribute to better understanding of the properties of potential solid-form candidates. “As a screening tool, high-throughput technology provides a platform to evaluate the potential for crystallizing new solid forms under certain conditions, albeit with some limitations. The information obtained solely from high-throughput technology is not sufficient to select a solid-form candidate for development, however,” he says. Consequently, rational experimental design with fit-for-purpose screening strategies based on rational screening or mixed screening approaches have become the norm for the industry, according to Selbo.
Large-scale screening is still employed as a part of intellectual property (IP) strategies for later-stage programs where there is more certainty of compound survival. “High-throughput screening is today an essential technology for mapping polymorphic forms, generating IP, and broadening the protected space in terms of polymorphs, and helping to avoid surprises as much as possible in advanced development phases,” Gil comments. For these programs, mixed screening services with rational, mid-, and high-throughput screens may be employed in an attempt to cover all bases, according to Cowans.
Sharp patterns
With respect to advances in technology for polymorph screening, Cowans points to improvements in x-ray powder diffraction (XRPD), which he refers to as the “quintessential tool for distinguishing different crystalline forms.” Most importantly, current-generation instruments are capable of providing the sharp, high-quality patterns that are necessary for identifying whether a material exists as a single crystalline phase and are amenable to indexing algorithms.
The combination of indexing software and advanced XRPD technology improves the ability to identify crystalline phases or mixtures, increases screening efficiency, and provides critical information about the various crystalline forms obtained, according to Cowans. SSCI has developed and patented its own indexing software specifically targeting organic molecules and integrated this tool into its routine screening activities.
Another important advance for polymorph screening is the reduction in the quantity of API required for analytical techniques (e.g., XRPD), according to Gil. Automation has also increased the efficiency of these analyses and accelerated sample pre-characterization.
Article Details
Pharmaceutical Technology
Vol. 40, No. 3
Pages: 32-35
Citation
When referring to this article, please cite it as C. Challener, ” Polymorph Screening for Identification of Relevant Crystalline Forms,” Pharmaceutical Technology 40 (3) 2016
Solid-State Nuclear Magnetic Resonance Spectroscopy for Analyzing Polymorphic Drug Forms and Formulations
 |
Scientists use several common techniques to analyze pharmaceutical solids. These methods, which include single-crystal X-ray diffraction, X-ray powder diffraction, differential scanning calorimetry, thermal gravimetric analysis, infrared, and Raman spectroscopy, have several advantages for the analysis of polymorphic drug forms and formulated products, but, in some cases, they can have some disadvantages (see sidebar, “Pros and cons of techniques for pharmaceutical solids analysis”).Solid-state nuclear magnetic resonance (SSNMR) spectroscopy has several advantages over these techniques. First, it is nondestructive and noninvasive. If little sample is available for analysis, scientists can acquire an SSNMR spectrum and return the sample for any further testing. SSNMR spectroscopy can be used on bulk drugs and on all types of drug formulations (e.g., tablets, capsules, suspensions, creams, and transdermal formulations) with little to no sample preparation. SSNMR spectroscopy is uniquely suited for testing drug formulations because the NMR resonances for most pharmaceutical excipients occur in a narrow range of the NMR spectrum. Thus, it is easy to distinguish the excipient from the active pharmaceutical ingredient (API) NMR resonances. Spectral subtraction can even be used to eliminate excipient peaks from the spectrum.
In addition, the technique can be used to study inclusion compounds such as those with cyclodextrins. Furthermore, the NMR spectrum can be used to examine host–guest interactions by monitoring changes in the NMR peak position and dynamics associated with a given nuclear site.
Moreover, SSNMR spectroscopy is quantitative and selective. Because NMR spectroscopy is inherently a quantitative technique (i.e., signal intensity is relative to the number of nuclear sites at that specific resonance frequency), SSNMR spectroscopy also can provide quantitative information if done properly, as will be discussed later in this article. This technique can quantify mixtures of polymorphic crystalline forms, or of crystalline and amorphous materials. Analyses can all be performed without the need for pure forms or a standard curve.The method also is selective; each NMR resonance seen in the NMR spectrum is caused by a specific nuclear site. Most often,13C NMR spectroscopy is used, but many other nuclei (e.g., 19F,15N, 23Na, 31P, 17O, or 2H) can be used, depending on what will be studied. NMR spectroscopy is unique because it can monitor a specific nuclear site. For example, it can be used to look for changes in the local chemical environment that may be caused by drug–excipient interactions or changes during the formulation process, along with the formation of different polymorphic or amorphous forms. In addition to the technique’s selectivity, isotopic labeling of low natural abundance nuclei such as 13C,15N, and 17O can be used to increase the sensitivity of a specific nuclear site. An example will be presented later in this article.
SSNMR spectroscopy also can be used to examine the structure of the material and its associated dynamics. For crystalline materials, one can determine the number of crystallographically inequivalent sites in the unit cell. It also can detect conformational changes and hydrogen bonding to some extent. Other, more advanced, two-dimensional techniques such as rotational echo double resonance can be used to measure distances (1). For amorphous materials, SSNMR spectroscopy can be used to examine the degree of disorder because various processing techniques (e.g.,lyophilization, spray drying, melt-quench, cryomilling) can vary the overall degree of disorder in the sample. |

Sometimes the system’s dynamics also can be used to investigate other aspects of the drug being studied. For crystalline polymorphic forms, some forms may be less stable than other forms, which could be caused by the overall molecular mobility. Correlations between the overall mobility or stability of polymorphic forms can be made by measuring the spin-lattice relaxation times (T1 and T1ρ). If a new form was discovered, the relaxation time could be measured to yield an early approximation of its overall stability. Sometimes, the same polymorphic form from different lots can exhibit different properties (e.g., stability, dissolution rate) which could be caused by the degree of crystallinity in the sample. The presence of defect sites or less-crystalline domains in the sample may not be observed in X-ray powder diffraction. This is not the case with SSNMR spectroscopy. These sites provide another avenue for the relaxation process of the spin states in the sample. Thus, a sample that has a lower degree of crystallinity would exhibit a faster spin-lattice relaxation time (T1), which may vary by less than a second to several seconds. This technique is not limited to analyzing only the bulk drug. It also can be applied to formulations. Changes in the overall dynamics of the drug during formulation could screen out potential drug formulations because of the increased mobility observed, possibly yielding a final candidate faster.
Despite its advantages over other common techniques, SSNMR spectroscopy also suffers from some disadvantages. It often requires a lot of expertise in the technique to run it properly. And, the equipment can be expensive, though most narrow-bore solution NMR spectrometers can be adapted to perform SSNMR work. Another obstacle is that the experiments are not routine (i.e., the experimental parameters used must be determined for a specific compound before running the NMR experiment). Automation is difficult because the sample often fails to spin when using an automatic program and requires human intervention. Compared with the techniques listed in the sidebar, “Pros and cons of techniques for pharmaceutical solids analysis,” SSNMR spectroscopy is the least sensitive and requires a large amount of sample to generate an adequate spectrum when studying low natural abundance nuclei such as 13C.
Analysis times for SSNMR experiments also can be problematic because they can range from a couple of minutes to a few hours, to a couple of days or more, depending on the sample being analyzed and what type of NMR experiment is used. Peak assignment in the SSNMR spectrum can be challenging because multiple peaks could be present for a single nuclear site or they may be overlapping peaks. Another problem is that the chemical shifts in the solid state can often differ as much as 10 ppm from the solution NMR values. A current review article shows many applications of this technique to the analysis of pharmaceutical solids (2).
General theory
Originally discovered in 1945, NMR involves transitions between nuclear spin states that become nondegenerate when placed in a magnetic field. The transitions resonate when an electromagnetic field at a specific frequency (i.e., based on the nucleus and magnetic field) is used.
In general, nuclei have a spin quantum number (I) which may be an integer, half-integer, or zero (not NMR active). A nucleus with integral or half-integer spin has an associated magnetic moment. When placed in a magnetic field, a torque is produced to align the magnetic moment with the magnetic field. Because this effect cannot occur, the torque produces a rotation about the axis of the magnetic field at a specific frequency of:

|
in which γ is the electromagnetic ratio for the given nucleus and B 0 is the magnetic field strength. If a second field, B 1, that is static and small is applied to the system, the nuclei nutate or wobble slightly in their orbits. But if the B 1 field oscillates at the same frequency as the nuclei, then the field will appear to be static to the nuclei and transitions between the spin states will occur. NMR spectroscopy is so powerful because each nucleus in the molecule is shielded by the surrounding electrons, so each nucleus sees an “effective field” that will change its resonance frequency. The frequency changes observed for the peaks in a molecule are reported in ppm to account for the differences in all of the magnetic fields that are used:
(shift in hertz [Hz]) ÷ (spectrometer operating frequency [Hz]) × 106 .
Two problems for performing NMR spectroscopy in the solid state are dipolar coupling and chemical shift anisotropy. Dipolar coupling arises because of the sample’s rigid nature. The equation for dipolar coupling is:
v = v a ± 0.5R(3cos2 θ–1)in which v is the resonance frequency,
for which γ is the magnetogyric ratio for the specific nucleus (A or X), r is the internuclear distance, μ0 is the permeability constant, and θ is the angle between the static magnetic field and the spin pairs.
One must be concerned with the γ and R in these equations. If a nucleus has both a high magnetogyric ratio and short internuclear distance, the dipolar coupling appears very large. For this reason, proton NMR spectroscopy is not performed in the solid state. For similar reasons, natural abundance also must be considered. With 13C at ~1% natural abundance, the probability of having two 13C nuclei next to each other in the molecule is very small, and thus only the heteronuclear dipolar coupling must be addressed. Normally, this dipolar coupling is ~50 kHz and is the strongest for CH and CH2; not as strong for CH3; and much weaker for quaternary carbons. To solve this problem and reduce or eliminate the dipolar coupling, a decoupling pulse is used. The decoupling pulse in SSNMR spectroscopy is similar to that used in solution NMR spectroscopy, but as much higher power (i.e., typically a few hundreds of watts). During the acquisition of the free-induction decay (FID), the radiofrequency (RF) amplifier for the nucleus to be decoupled is turned on. This causes the spins to be flipped rapidly between spin states, thus stopping the dipolar-coupling spin-exchange process and thereby narrowing the resonances (see Figure 1).
Chemical shift anisotropy (CSA) is another main problem. The NMR spectrum of a solid sample can be very broad because of the individual crystallites’ multiple orientations in the magnetic field. The equation for chemical shift anisotropy is:
|
in which σ is the observed chemical shift, σiso is the isotropic chemical shift, σaniso is the anisotropic chemical shift, and θ is the angle between the shielding vectors and the magnetic field. To get high resolution NMR data in the solid state, only the isotropic chemical shift must be obtained. For this to occur, the anisotropic portion must be zero and can be accomplished by setting the angle to 54.7°. By rapidly spinning the sample about this axis (i.e.,magic-angle spinning), the anisotropic portion cancels out thereby giving high-resolution spectra (see Figure 1). Spinning speeds can be approximately a few kHz to 35 kHz or more (i.e.,180,000–2,100,000 RPM or more). The 54.7° angle is also the angle of the body diagonal of a cube. By spinning about this angle, each crystallite spends an equal amount of time in the x, y, and z dimensions, thereby averaging out the crystallites’ multiple orientations in the sample. If the spinning rate is not fast enough, artifacts, called spinning sidebands, will appear at integral factors of the spinning speed (see Figure 1, only the peaks at ~17 and ~133 ppm are real). Based on the spinning rate compared with the width of the static NMR powder pattern, a certain percentage of the main peak intensity will be lost in the spinning sidebands. This point is important if quantitation is to be performed.The spinning sidebands can be eliminated in two ways. First, the sample can be spun faster than the width of the static powder pattern, which could be as high as 50 kHz or more. Second, a special pulse sequence can be used to cancel out the sidebands. More information about this process will be presented later in this article.
Common and advanced techniques
With 13C SSNMR spectroscopy, the recycle delay between transients can range from a few seconds to an hour or more. For this reason, cross polarization (CP) is often used.With 1H- 13C CPMAS NMR spectroscopy, relaxation times are not dictated by the 13C relaxation rate, but rather by the 1H relaxation, which is much shorter in most cases. A typical CP experiment consists of four main steps: generate the magnetization on the abundant nuclei (1H), transfer this magnetization to the dilute nuclei (13C), acquire the dilute spin (13C) FID, and decouple the abundant spin (1H). The magnetization from the 1H is placed on the x-axis by applying a π/2 or a 90° pulse. This procedure is followed by another pulse, which is sometimes termed the contact pulse, contact time, or the Hartman–Hahn matching condition.
The process of transferring the magnetization from the abundant to dilute spin is accomplished through the use of the heteronuclear dipolar interactions by a process of spin exchange. This will only work if the nuclei are at the same resonance frequency. Because the main magnetic field cannot be changed, the B 1 field must be changed. When the B 1 field is applied at the resonance frequency of the specific nuclei, the nuclei will precess as if they were the only applied field. For 1H- 13C CP, if γH B IH = γC B IC, the Hartman–Hahn condition is achieved. |

Figure 1: Comparison of the13C NMR spectra of hexamethyl-benzene acquired using several techniques. DD is dipolar decoupling, MAS is magic-angle spinning, and CP is cross polarization.
Often, the duration of the contact pulse must be optimized because two main factors affect the signal intensity: the cross polarization time constant (TCH) and the proton spin-lattice relaxation in the rotating frame (T1ρH). Initially, the magnetization builds up because of the TCH, and then the T1ρH will cause the magnetization to decrease. Thus, a contact time period must be chosen that allows for the maximum magnetization transfer or maximum signal intensity. Not all samples will exhibit the same CP profile. Some samples may have a very fast TCH while others are slow. The same scenario applies to the T1ρH. Thus, quantitation by CPMAS NMR spectroscopy must be performed very carefully. Another benefit of CP is that the sensitivity is increased by a factor based on the magnetogyric ratios of the two nuclei. The factor is 4 for 1H- 13C and 10 for 1H- 15N.
Another pulse sequence addition is that of total sideband suppression (TOSS). With complex NMR spectra, the presence of spinning sidebands can cause problems for determining which peaks are real and which are artifacts without conducting multiple spinning speed studies. The sequence consists of four 180° pulses in which the sideband phases are modulated. The times between the pulses—based on the rotor period (τR)—are set so that the centerband is refocused and the sidebands cancel. A visual representation of the pulse sequence is shown in Figure 3 with an example spectrum.A couple of advanced CP techniques such as variable amplitude cross polarization (VACP) and ramped CP also can be used. These techniques are needed because of the rapid spinning rates that are used in modern SSNMR systems. Because CP transfers magnetization through dipolar couplings, the dipolar couplings are partially averaged at fast spinning rates (i.e., the equation for dipolar coupling has the same angular dependence as the chemical shift anisotropy [3cos2 θ – 1]). This will lower the overall CP efficiency, hence yielding lower sensitivity. In both VACP and ramped CP, the intensity of the B1H field is varied, thereby transferring the magnetization that would have been lost in the spinning sidebands. Figure 2 offers a visual representation of the standard CP pulse sequence along with the VACP and ramped CP pulse sequences.

Figure 2. Schematic representation of cross polarization (CP) pulse sequences. X) X channel (i.e., 13C, 15N), a) standard CP, b) ramped CP, c) variable amplitude CP. 1 is 1H 90° pulse, 2 is the contact pulse, 3 is the1H decoupling pulse.
This pulse sequence has a few disadvantages. The sideband intensity is not refocused into the centerband. If the spectrum was acquired at a low spinning rate, the centerband intensity can be greatly reduced. Another problem is that if the four 180° pulses are not perfect, then additional artifacts will be present in the spectrum.
Component identification
With SSNMR spectra, there are some general regions that can be used to aid in the assignment of a spectrum (see Table I). For the drug itself, the spectra can vary, but they mostly fall in the same regions because of the common functional groups. Most often, the API consists of aromatics, aliphatics, carboxylic acids, and heteroatoms. Excipients usually will not have any aromatics, aliphatics, or carboxylic acids, thus allowing for the easy identification of these elements in the NMR spectrum (see Figures 4 and 5).
But, this is not the case for XRPD. The diffraction lines can occur at any location, which can make component identification difficult in a formulated product (see Figure 5). To show this effect further, several blends of ranitidine HCl (Alfa Aesar) and lactose monohydrate (USP) were prepared and analyzed by XRPD and SSNMR spectroscopy.
When using a standard scan rate of 1°/min, a detection limit of ~5% was achieved (see Figure 6). Many diffraction lines overlapped, thus further limiting the detection of small amounts of ranitidine HCl. This was not the case with the SSNMR experiments (see Figure 7). Using this method for the analysis (8-h experiment time), the peaks were clearly resolved and a detection limit of ~0.5% was calculated based on the signal–noise ratio. It is interesting to note that the lactose peaks in the blends prepared were not as intense as they should have been, based on the percentage of lactose present. This was because the NMR experiments were conducted with optimized parameters for the ranitidine HCl (recycle delay and contact time). With excipients, the relaxation time usually is much longer. For example, for lactose at 9.4 Tesla, the relaxation time was ~200 s, whereas the relaxation time for ranitidine HCl was 10 s. Because of this, the lactose peaks became saturated over the course of the NMR experiment and did not add any signal to the peaks.
Figure 3. Schematic representation of a variable amplitude cross polarization (VACP) pulse sequence with total sideband suppression (TOSS) and corresponding changes observed in the 13C CPMAS NMR spectra of neotame monohydrate.

Figure 4. 13C CPMAS spectra comparing a commercial ibuprofen tablet and ibuprofen.

Figure 5. 13C CPMAS spectra and X-ray powder diffraction profiles of some common excipients.

Figure 6. Determination of the detection limit of ranitidine HCl in a lactose blend by XRPD. Arrows indicate resolved diffraction lines.
In addition, the blend had only two components. If more excipients were added, the detection limit by SSNMR spectroscopy would remain essentially the same because the excipient peaks fall in the same region of the NMR spectrum. If any inorganic excipients are used, they will not even be seen in the NMR spectrum. In the case of XRPD, this cannot be known.
Another interesting use for SSNMR spectroscopy is to determine pH (3). In a solution, only a standard pH meter is required. In the case of solids, no current analytical method can determine pH either in a formulation or during stability testing. This is important if the compound either undergoes a hydrolysis reaction or is sensitive to changes in the pH. It is possible to perform the measurement with SSNMR spectroscopy. This can be done by the use of a probe molecule, which can be any weak acid or base that has a pKa(s) in the range being examined. This probe molecule also must be 13C enriched ensuring that only 1 to 2 mg is added to 1 g of the total sample so that it will not have any other effect on the product. For example, fumaric acid is readily available, 13C enriched, and has two pKa values (3.03, 4.54) for a wider range of pH values (4).
 Figure 7. Determination of the detection limit of ranitidine HCl in a lactose blend by 13C CPMAS nuclear magnetic resonance spectroscopy. |
The relative concentration of the species present with respect to pH shows that, depending on the detection limit of the salts produced, a 1.5–6.5 pH range may be possible (see Figure 8). To determine the pH relating back to a standard pH scale, a standard curve must be determined. This is done by dissolving some standard fumaric acid and adjusting the pH of the resulting solution. Because the solid must be isolated at this specific pH, the sample must be flash frozen to “lock” the molecular state. Then, the frozen solution is lyophilized to get the solid for analysis by SSNMR spectroscopy.
 Figure 8. Concentration profile and13C CPMAS NMR spectra at designated pH for fumaric acid. |
Based on the data in Figure 8, fumaric acid can be used to monitor the pH from ~2 to 5 (5). At low pH, only a single carbonyl peak is observed. When the pH is increased, however, other peaks are seen because of salt formation. By plotting the relative values of these peaks at each pH, a standard curve can be prepared and then related back to the sample to yield the pH. The lower and higher pH values were hard to determine because the optimized NMR values for generating the standard curve were those of the stock fumaric acid. This is also why the peaks’ relative intensities in the NMR spectrum do not match the theoretical calculations. This is necessary because all NMR experiments for preparing the standard curve and the sample must be measured using identical parameters (except for the number of transients).

Figure 9. 13C CPMAS spectra of13C labeled aspirin, a) 13C CPMAS spectrum of the sample, b)13C CPMAS spectrum of aspirin (natural abundance), c) result of spectral subtraction showing only the 13C labeled carbon.
With the use of selective isotopic labeling, the analyst can monitor very low levels of any impurity, either polymorphic, degradation product or generation of amorphous material. Because the natural abundance of 13C is 1%, isotopic labeling can increase the sensitivity of a single nuclear site by as much as two orders of magnitude. Care must be taken if the entire molecule will be 13C labeled because this will introduce 13C– 13C dipolar interactions, thereby decreasing the overall spectral resolution (6).
Figure 11. 13C CPMAS spectrum of a) aquired after stability testing, b) after drying the poststability sample, c) obtained after spectral subtraction of 13C CPMAS NMR spectrum of natural abundance aspirin (*) amorphous aspirin, (**) amorphous salicylic acid.
Researchers have examined drug degradation in the solid state by using aspirin as a model (7). Aspirin was chosen because it has been well characterized and it is easily synthesized with13C isotopic enrichment on the carbonyl carbon. It also is representative of drug degradation by hydrolysis. Some initial work was performed that showed little to no degradation when crystalline aspirin was placed in the stability chamber and subsequently analyzed by HPLC. The drug was pure crystalline, however, and may not be representative of a manufactured tablet. Studies were conducted to examine highly energetic states in the presence of a bulk, less reactive state. Highly energetic states may be formed during the formulation process, and the formulation’s overall stability may be governed by these small amounts of highly energetic states. Samples were prepared by either grinding or freeze-drying 13C labeled aspirin with hydroxy-propylmethylcellulose (HPMC) (6 mg13C labeled aspirin/100 mg HPMC) and then adding it to 294 mg of crystalline aspirin (1.5% impurity level for 13C labeled aspirin). These samples were then placed in a stability chamber at 70° C, 50% RH for seven days and analyzed by SSNMR spectroscopy. Spectral subtraction was used to isolate the NMR peak easily because of the 13C labeled site from the bulk aspirin (see Figure 9). For the formulation blends shown previously, the peaks caused by HPMC did not interfere with any of the aspirin peaks. For the ground samples, no degradation products were observed. The NMR peak of the labeled material decreased in intensity. A decrease in the cross-polarization efficiency caused this effect because the sample was “wet.”

Figure 10. 13C CPMAS spectra of a) 13C labeled aspirin after freeze drying with HPMC, b) blend with natural abundance aspirin, c) acquired after stability testing.
PolymorphismWith the freeze-dried sample (see Figure 10), the initial sample was amorphous aspirin, which would be expected to be highly reactive. After seven days, however, no peaks from the labeled component were seen. After seven days, however, no peaks from the labeled component were seen. After the sample dried overnight in a 50° C oven and was analyzed by SSNMR spectroscopy, the degradation product peak was observed, which was that of amorphous salicylic acid (see Figure 11). This finding was not expected because it was anticipated that amorphous aspirin would degrade and recrystallize to a crystalline form of salicylic acid. This low level of impurity would have been seen easily when performing an HPLC assay, however it would not have been able to determine whether the impurity was amorphous. It is likely that SSNMR spectroscopy would be the only technique to possibly answer this question, especially at only a ~1% level.

Figure 12. Crystal struture and 13C CPMAS NMR spectra of pseudopolymorphs of nedocromil Na with tentative spectral assignments.
Polymorphism, or the ability of a substance to adopt two or more configurations or arrangements in the crystal lattice, is a concern whenever a solid dosage form is used. Through either the manufacturing of the API or the formulation process, polymorphs can be generated. Most often, XRPD is the technique of choice for the identification of polymorphic forms because it is a readily available technique. As shown in previous examples, if a polymorphic change occurs during the formulation process, the detection limit may not be that great using XRPD. SSNMR is capable of detecting different polymorphs easily. NMR spectroscopy, unlike XRPD, is sensitive to changes in the local chemical environment, thus slight changes in bond lengths, bond angles, interactions with neighboring molecules or, in the case of pseudopolymorphs, different salts, hydration levels, or solvates can have an effect on the NMR spectrum. The changes seen in the chemical shift can be affected by several factors. The gamma gauche effect can result in a significant change in the chemical shift. Isotactic polypropylene results in a 10-ppm shift from syndiotactic polypropylene. In addition, an eclipsed conformation is ~5 ppm different than a staggered conformation, which is most significant for aliphatic carbons. Conjugation in the molecule is also significant with molecules containing double bonds or carbonyl groups attached to aromatics. The overall amount of conjugation is often determined by the relative orientation of the groups and can cause a 15 ppm shift or more. Some pseudopolymorphs of nedrocromil salts are shown in Figures 12 and 13 (8). As Figure 12 shows with the two sodium salts, a change occurred in the orientation of the carbonyl group attached to the aromatic carbon, resulting in approximately an 8-ppm shift in the chemical shift of the quaternary carbon (No. 8) that is easily resolved. This same quaternary carbon shows similar changes for the pseudopolymorphs analyzed allowing for good resolution at determining the form present. Another possible change is a difference in the arrangement of the molecules in the crystal lattice. This type of change usually will result in a change in chemical shift of 1 to 2 ppm. Because it is difficult to find a system that has a change in the arrangement in the crystal lattice without a corresponding change in the molecular conformation, it is difficult to determine what the actual chemical shift change will be.
Figure 12. Crystal struture and 13C CPMAS NMR spectra of pseudopolymorphs of nedocromil Na with tentative spectral assignments.

Figure 13. 13C CPMAS NMR spectra of some pseudopolymorphic forms of nedocromil salts.
Possibly the most important application of SSNMR spectroscopy is quantitation. NMR spectroscopy is inherently quantitative because the signal’s intensity is directly proportional to the number of nuclei at that given resonance frequency. This is not the case when cross polarization is used. Because most 13C SSNMR spectra are acquired using CP, this must be considered before performing any type of quantitation work.
Figure 14. 13C CPMAS NMR sp
ectra of anhydrous neotame a) Form G, b) Form A, c) amorphous. Asterisk indicates quaternary aromatic peak used in quantitation.
Three main items must be accounted for: the spin-lattice relaxation time for the proton (T1H), the cross polarization constant (TCH), and the spin-lattice relaxation time in the rotating frame for the proton (T1ρH). First, for accurate quantitation, the relaxation time should be equal to or greater than five times the longest T1 of the components present in the sample. This factor will ensure that all of the spin states have returned to equilibrium. If the relaxation delay is too short, then the quantitation values will be off (because of partial saturation of the peaks with the longest T1 value).

Figure 15. Plot of integrated intensities versus contact time obtained when analyzing a 50% (wt–wt) mixture of anhydrous neotame Form A and Form G.
Another problem is a combination of TCH and T1ρH. If magnetization is not allowed to be transferred fully or if the decrease in magnetization varies for the components in the mixture, the results will be incorrect. For example, neotame, a newly developed sweetener, has multiple polymorphic forms. Form A and G and amorphous material have been quantitated with relatively good accuracy by SSNMR spectroscopy (see Figure 14) (9). In the case of the crystalline polymorphs A and G, the TCH times were nearly identical, however the T1ρH times vary significantly. To account for the decrease in magnetization over time, a multiple contact time approach was used. NMR experiments were conducted at multiple contact times and were fit (see Figure 15). By using this fit and extrapolating back to a contact time of zero, researchers accounted for the decrease in intensity over time by only using an equilibrium magnetization intensity. The results from this work and associated errors are presented in Table II.
With the amorphous material and either crystalline polymorph, there were many errors with the individual quantitation results for each mixture. Nonetheless, when comparing a plot of the measured with actual amorphous content, the data fit well, leading to the discovery of the presence of amorphous material (<20%) in the crystalline samples. For the NMR spectrum of an amorphous material, peaks are roughly ten times as broad and based on the positions of these amorphous peaks relative to the crystalline peaks, the amorphous peaks sometimes will appear to blend in with the baseline. Additional experiments were conducted that proved the presence of amorphous material in the crystalline sample.
Because amorphous peaks generally are very broad, the detection limit for an amorphous material is ~10–20%, based on the relative location of the amorphous and crystalline peaks in the spectrum. Nonetheless, one can increase the detection limit and even perform quantitation on samples with small amounts of amorphous material. First, amorphous materials generally have T1H times that are much faster than the crystalline forms. Thus, the NMR peaks from the crystalline material can be attenuated by using a relaxation time (or contact time) that is optimized for the amorphous material, thereby saturating or reducing the relative intensity of the crystalline peaks. As for the quantitation of low amounts of amorphous material, a standard addition method can be used if amorphous material can be made and verified to be amorphous by SSNMR spectroscopy. If amorphous material stability is an issue, the NMR experiments can be run at a low temperature to limit any degradation or recrystallization during the course of the NMR experiment. For the actual quantitation experiments, a single sample must be prepared with a known amount of amorphous material added to the sample. After the NMR experiments are completed, the percentage of amorphous material in the original sample can be calculated easily. If a very small amount of amorphous material is expected in the API, multiple quantitation experiments should be performed to generate some statistics to go along with the determined amorphous content.

Table II: 13C CPMAS NMR quantitation results of anhydrous neotame polymorphs A and G.
Conclusions
Solid-state nuclear magnetic resonance (SSNMR) spectroscopy is an excellent technique for performing many different types of analyses on pharmaceutical solids. It has some disadvantages, but it also has many advantages over other techniques. Though SSNMR spectroscopy is often considered a “last chance” technique, it should be considered seriously as the technique of choice if there are doubts about other current analytical techniques’ ability to achieve the necessary results.
References
1. F.G. Vogt et al., “Determination of Molecular Geometry in Solid-State NMR: Rotational-Echo Double Resonance of Three Spin Systems,” J. Phys. Chem. B 107 (5), 1272–1283 (2003).
2. P.A. Tishmack, D.E. Bugay, and S.R. Byrn, “Solid-State Nuclear Magnetic Resonance Spectroscopy—Pharmaceutical Applications,” J. Pharm. Sci. 92 (3), 441– 474 (2003).
3. B. Henry, P. Tekely, and J.-J. Delpuech (2002). “pH and pK Determinations by High-Resolution Solid-State 13C NMR: Acid-Base and Tautomeric Equilibria of Lyophillized L-Histidine,” J. Am. Chem. Soc. 124 (9), 2025–2034.
4. S. Budavari et al., Eds., The Merck Index, (Merck Research Laboratories, Whitehouse Station, NJ, 1996).
5. J. W. Lubach et al., unpublished results.
6. M.T. Zell et al., “Two-Dimensional High-Speed CP/MAS NMR Spectroscopy of Polymorphs 1. Uniformly 13C-Labeled Aspartame,” J. Am. Chem. Soc. 121 (6), 1372-1378 (1999).
7. B. Chen et al., “Monitoring the Reactivity of Amorphous Aspirin as a Minor Component with 13C Isotopic Labeling and Solid-State 13C CPMAS NMR Spectroscopy,” paper presented at the American Association of Pharmaceutical Scientists National Meeting, Salt Lake City, UT, Oct. 26–30 2003.
8. L.R. Chen et al., “Nuclear Magnetic Resonance and Infrared Spectroscopic Analysis of Nedocromil Hydrates,” Pharm. Res. 17 (5), 619–624 (2000).
9. T.J. Offerdahl et al., “Quantitation of Crystalline and Amorphous Forms of Anhydrous Neotame Using 13C CPMAS NMR Spectroscopy,” J. Pharm. Sci. 94 (12), 2591–2605 (2005).
New FDA Guidance on Polymorphic Compounds in Generic Drugs
R
ockville, MD (July 9)—A new guidance issued by the
US Food and Drug Administration earlier this month advises companies on how to treat polymorphic drug compounds—those that exhibit multiple structural forms—in filing abbreviated new drug applications (ANDAs). The bottom line, according to the guidance, is that generic drug products containing the polymorphs be the “same” as the reference listed drug (RLD) in active ingredients, bioavailability, and bioequivalence. The guidance pertains to orally available drugs that are either solid- or suspension-dosage products.
Polymorphisms arise when compounds are identical chemically, but not structurally. This can happen when two solids take on different crystalline forms—such as graphite and diamond; when molecules are disordered and fail to produce a repeatable crystal lattice, as is the case for the molecules in glass; or when solvent is trapped inside the crystal structure—as in hydrates, where water molecules are found within crystals. The guidance notes that different polymorphisms may alter physical properties of compounds and affect their solubility, which in turn can alter their bioavailability or bioequivalence. In addition, polymorphic forms of a compound may alter the way the compound behaves during production, which again, may alter the finished drug’s biological activities. On this latter point, the guidance specifically states, “Since an ANDA applicant should demonstrate that the generic drug product can be manufactured reliably using a validated process, we recommend that you pay close attention to polymorphism as it relates to pharmaceutical processing.”
The guidance also emphasizes the effect polymorphisms may have on drug stability, which again, may alter the drug’s biological activity. �But the guidance goes on to say that “it is the stability of the drug product and not stability of the drug substance polymorphic form that should be the most relevant measure of drug equality.” Otherwise, a generic drug can be considered the “same” as the active ingredient in an RLD if the generic compound conforms to the standards set out in a United States Pharmacopeia (USP) monograph, if one exists for that particular drug substance. These standards generally include the chemical name, empirical formula, and molecular structure of the compound. However, the “FDA may prescribe additional standards that are material to the sameness of a drug substance.” But as concerns polymorphisms, the guidance goes on to say “…differences in drug substance polymorphic forms do not render drug substances different active ingredients for the purposes of ANDA approvals….” Finally, the guidance reminds ANDA applicants that the biological performance characteristics of a drug are also dependent on the drug’s formulation and advises applicants to consider the properties of both the drug substance and formulation excipients, when assessing “sameness.” For the full guidance, click here.
Cocktail-Solvent Screening to Enhance Solubility, Increase Crystal Yield, and Induce Polymorphs
Crystallization from solutions is not only an important step in the fabrication of various functional materials in biological systems, but also a key separation and purification process in the manufacture of many fine chemicals and specialty chemicals, especially pharmaceuticals (1, 2). Pharmaceutical crystallizations are often carried out in batches of organic solvents or mixtures of solvents through temperature cooling (3). Because of the excess properties (i.e., the difference between the real properties and the ideal properties) for a real solution, the solubility of an active pharmaceutical ingredient (API) in a solvent mixture is sometimes higher than its solubility in a single solvent as the activity coefficient decreases (4, 5). The solubility enhancement that the solvent mix offers can bring three main advantages to pharmaceutical batch crystallization:
- It replaces excellent but environmentally harmful solvents such as tetrahydrofuran (THF), N, N-dimethylformamide (DMF), and N, N-dimethylacetamide (DMA) with greener solvents such as alcohols, acetonitrile, and water
- It gives a higher crystal yield
- It increases the degree of supersaturation, thus allowing nucleation to be controlled by the lowest nucleation barrier so that the metastable, instead of the thermodynamically stable crystalline phase, is induced (6–8).
Binary-solvent mixtures result when a second solvent (i.e., an antisolvent or cosolvent) is added to a subsaturated solution until the degree of supersaturation is high enough for crystallization to take place under isothermal conditions. Performing further crystallization in ternary solvent mixtures may be a new method of influencing the nucleation rate, the shape of the product crystals, the size distribution of the entire crystallized mass, aggregate and agglomeration properties, the purity of the crystals, and polymorphism (9–14).
Because solubility, crystal yield, and polymorphism are solvent dependent, solution recrystallization by solvent screening is of fundamental and of foremost importance to many chemical process industries. The process is especially important for manufacturing APIs (15–22). Pharmaceutical companies have a limited amount of time and materials to advance new chemical entities from candidacy to Phase I clinical trials (23).
The aim of this article is to extend initial solvent screening for a single-solvent system to the cocktail solvent screSulfathiazole, a valuable antimicrobical sulfa drug used in veterinary medicine (see Figure 1), was chosen for this study mainly because of its commercial value and its five polymorphs, which are well-characterized by Fourier-transform infrared spectroscopy (FTIR), powder X-ray diffraction (PXRD), and differential scanning calorimetry (DSC) (10, 15, 17).Under the initial solvent-screening strategy of single solvent systems, 24 solvents, mostly useful for scale-up, were selected (25). If all 24 solvents had been taken into account, the possible combinations for binary and ternary solvent mixtures would have been 24! ÷ (22!2!) = 276 and 24! ÷ (21!3!) = 2024, respectively. However, for the purpose of showing the feasibility of cocktail-solvent screening, the authors limited the screening to three miscible green solvents (acetonitrile, n-propanol, and water) and their combinations. N-propanol and acetonitrile produce Form I and Form IV sulfathiazole crystals, respectively. Water is a common solvent in the manufacture of sulfathiazole (15, 26). |

At first, the solubility of sulfathiazole in each solvent or combination of solvent mixtures at 15, 25, 40, and 60 °C was measured by gravimetric titration (see Figure 2). This method did not require any calibration and was more robust than weighing the dry-residue mass, which might prompt the formation of sulfathiazole solvates during the drying process (11, 19). The crystal yield was estimated by finding the solubility difference between 60 and 25 °C for each solvent or solvent mixture. Solid generation of sulfathiazole in each solvent or solvent mixture was then achieved in a 20-mL scintillation vial by gentle shaking and cooling from 60 °C in a water bath to 25 °C in another water bath. The cooling rate of a solution with a volume of less than 20 mL was almost independent of the volume and the nature of a solvent. The cooling profile could be approximated by an exponential decay determined experimentally as:
T = 26 + 31 exp (-t ÷ 0.9)
where T = temperature (°C) and t = time (min). The relatively rapid decrease in temperature served as an ideal way to preserve kinetically induced polymorphs by a sudden surge of supersaturation (8). Solids produced were vacuum filtered at once and oven dried at 40 °C for 4 h. DSC and thermogravimetric analysis (TGA) were used mainly to determine the polymorphism of sulfathiazole solid samples. Optical microscopy (OM) was used for crystal-habit imaging.
Materials and methods
Active pharmaceutical ingredient. Sulfathiazole (Form III) white crystalline powders (C9H9N3O2S2, MW: 255.31, m.p. = 201–204 °C, 98%, Lot: 410504/1 51804006) were purchased from Fluka (Buchs, Switzerland).Solvents. Table I lists the solvents used in this study and the companies that provided them. Reversible osmosis (RO) water was clarified with a water purification system (Milli-RO Plus, Millipore, Billerica, MA).
Solubility and crystal-yield studies. About 10 mg of sulfathiazole Form III crystals were weighed in a 20-mL scintillation vial. Drops of solvent or solvent mixture were titrated into the vial carefully by micropipette and shaken intermittently until all sulfathiazole Form III solids were dissolved. The solubility of sulfathiazole Form III solids at a given temperature was calculated as the weight of sulfathiazole Form III solids in a vial divided by the total volume of solvent or solvent mixture added to a vial. The solubility of sulfathiazole Form III solids in the same solvent or solvent mixture at 15, 25, 40, and 60 °C was determined. The crystal yield was calculated as the difference between the solubility at 60 °C and at 25 °C. All temperatures were maintained and controlled by a water bath. Despite the inherent inaccuracy (±20%) of measuring volume by sight, this method provided a rapid and robust technique for process scale-up (24).
Solvent-miscibility studies. Of the 24 solvents, about 1-mL portions of each solvent in a pair were shaken together for approximately 1 min in a 20-mL scintillation vial at 25 °C at 1 atm. Assuming that solvent miscibility was independent of the weight fraction of solvent, the solvent pair was considered to be miscible if no interfacial meniscus was observed after the contents of the vial were allowed to settle. If a meniscus was observed without apparent change in the volume of either solvent, the pair was regarded as immiscible (24).

Table I: Sources of solvents used in this experiment.
Polymorph studies. A saturated sulfathiazole solution of a particular solvent or solvent mixture was prepared in a 20-mL scintillation vial in accordance with the solubility values determined at 60 °C from the experiments in “Solubility and crystal-yield studies” above. Solids were generated by cooling from 60 °C in a water bath to 25 °C in another water bath and intermittent shaking. Solids were immediately vacuum filtered and oven dried at 40 °C for 4 h. Polymorphism and crystal habits were determined by DSC, TGA, and OM.
DSC. A differential scanning calorimeter (DSC 7, Perkin Elmer, Norwalk, CT) was used to monitor thermal events during heating. The instrument was calibrated with indium (8–10 mg, 99.999% pure, extrapolated melting onset at 156.6 °C). All samples were run in crimped aluminum pans under a constant nitrogen purge. Each sample was heated at 10 °C/min.
TGA. A thermogravimetric analyzer (TGA 7, Perkin Elmer) was used to measure changes in the weight of a specimen while varying temperature in a controlled nitrogen atmosphere. About 2 to 3 mg of sample were placed on an open platinum pan and heated at 10 °C/min.
OM. An optical microscope (SZII, Olympus, Tokyo, Japan) equipped with a charge-coupled-device camera (SSC-DC50A, SONY, Tokyo, Japan) was used to take images of crystal habit.
Results and discussion
The 24 solvents, arranged in ascending order by their total Hildebrand values, were n-heptane, xylene, p-xylene, ethyl acetate, toluene, methyl tert-butyl ether (MTBE), benzene, methyl ethyl ketone (MEK), chloroform, THF, DMA, acetone, 1, 4-dioxane, nitrobenzene, n-butyl alcohol, isopropyl alcohol (IPA), benzyl alcohol, acetonitrile, n-propanol, DMF, ethanol, dimethyl sulfoxide (DMSO), methanol, and water (24).
Sulfathiazole Form III crystals dissolved well in 11 solvents (labeled “good solvents”): MEK, THF, acetone, benzyl alcohol, acetonitrile, n-propanol, DMF, ethanol, DMSO, methanol, and water. The crystals dissolved only slightly in 13 solvents (labeled “bad solvents”): n-heptane, xylene, p-xylene, ethyl acetate, toluene, MTBE, benzene, chloroform, DMA, 1, 4-dioxane, nitrobenzene, n-butyl alcohol, and isopropyl alcohol. The crystals’ solubility in these solvents was < 0.5 mg/mL at 25 °C.
Based on the solvent-miscibility studies of the solvent pairs of the 24 solvents, there were 38 gray boxes ÷ 2 = 19 immiscible pairs (because of the symmetry in Table II). The pure-solvent systems are represented by the 24 diagonal boxes in Table II. The form space (i.e., a probable condition of discovering a new polymorph) of the pure-solvent systems for our initial solvent screening was limited to the number of good single solvents, represented by the yellow boxes in Table II (24). Therefore, the probable condition of discovering a new polymorph for sulfathiazole was 11. However, if the good cosolvent systems (i.e., binary miscible mixtures of good solvents) are taken into account, the form space would be extended to the number of blue boxes in Table II ÷ 2 = 54. In addition, if the antisolvent systems (i.e., binary miscible mixtures of a good and a bad solvent) are also considered, the form space of the antisolvent systems would be calculated as the number of green boxes in Table II ÷ 2 = 126.
Currently, the total form space should then be at least equal to 11 + 54 + 126 = 191. The total form space is expected to expand dramatically if various solvent compositions of binary mixtures, temperatures, and ternary solvent systems are also considered (14). Solid generation by cooling can be applied in the yellow and blue regions in the form space (see Table II) if solubility curves are available. However, isothermal condition is usually employed if solid generation is achieved by adding antisolvent to the green domain (see Table II). Generally, no attempts are made to generate solids in the regions of immiscible solvent pairs (gray boxes), bad solvents (red boxes), and cosolvents of bad solvents (white boxes) (i.e., binary mixture of miscible bad solvents) in the form space (see Table II).
Since the 24 × 24 form space in Table II did not take the solvent compositions and the ternary solvent mixtures into account, the authors developed a “triangular form space” for given ternary solvent mixtures. Three of the 11 good solvents (acetonitrile, n-propanol, and water) were used to concoct three different binary mixtures and 10 different ternary mixtures (see Figure 2). Consequently, there were 3 + 10 = 13 combinatorial mixtures in total. The compositions of each mixture were expressed by mole fraction ratios of acetonitrile:n-propanol:water. The 13 points and three single solvents were coordinated on a triangular graph (see Figure 2). The corresponding 16 solubility curves of sulfathiazole Form III crystals were constructed and grouped by their solubility ranges at 15, 25, 40, and 60 °C for ease of comparison (see Figure 5). Solubility curves at 15, 25, 40, and 60 °C for the binary and ternary mixtures as a function of mole fractions are illustrated in Figure 6. The crystal yield, based on the solubility difference from 60 to 25°C in Figure 5, is shown in Figure 7. The crystal yields of the solvent mixtures such as (80:10:10), (60:20:20), (45:10:45), and (50:0:50) were much higher than that of acetonitrile, n-propanol, and water individually, and almost as high as that of THF.Therefore, only 11 solubility curves of sulfathiazole Form III crystals in 11 kinds of good solvent, based on their solubility at 15, 25, 40, and 60 °C, were constructed and grouped by their solubility ranges for ease of comparison (see Figure 3). The crystal yield was calculated as the solubility difference between 60 and 25 °C, based on Figure 3 for each solvent (see Figure 4). Although solvents such as DMF and DMSO gave high crystal yields, they are environmentally harmful (6).

Table II: The 24 3 24 form space of sulfathiazole Form III crystals at 25 8C.


Solids generated by the cooling method from the 11 good solvents (i.e., the yellow boxes in Table II) and 13 solvent mixtures (i.e., points 4–16 in Figure 2) were isolated and then analyzed by DSC and TGA. Although sulfathiazole forms solvates, TGA showed that none of the solids were solvates or hydrates because no weight loss occured from 50 to 205 °C for all solids (19). Based on the five general melting points of Forms I–V sulfathiazole crystals at around 203, 198, 175, 166 (broad), and 148 °C (broad), respectively, DSC responses in Figure 8 illustrated the various sulfathiazole crystal forms grown in the 11 single solvents: MEK (Forms I, III, and V), THF (Form III), acetone (Forms I and V), benzyl alcohol (Form I), acetonitrile (Forms I, III, and IV), n-propanol (Forms I and V), DMF (Form III), ethanol (Forms I, III, IV, and V), DMSO (Forms I, III, and V), methanol (Forms I, III, and V), and water (Forms I and III) (15).Comparing the solubility of sulfathiazole Form III crystals in each solvent at 25 °C (see Figure 3) with the solvent properties, the authors found that the dispersion-force contribution (Hansen dispersion parameter d) was small in all cases, but the polar-force contribution (Hansen polar parameter p) and the hydrogen-bonding contribution (Hansen hydrogen-bonding parameter h) were critical (24). The solvent needed relatively strong polar force, hydrogen bonding, or both to achieve acceptable solubility by disrupting the hydrogen-bonded network in crystalline sulfathiazole (9). This criterion is shown by the fact that the highest solubility was about 0.03 g/mL sulfathiazole Form III crystals in ternary solvent mixtures of acetonitrile, n-propanol, and water with mole fraction ratios of (45:10:45) (see Figure 6e) and (50:0:50) (see Figure 6a) at 25 °C. Acetonitrile and water individually had high values of 18.0 MPa¼ and 42.3 MPa¼ for δp and δh at 25 °C, respectively (24). Only when they were both blended at equally high mole fractions could the resultant mixed-solvent system exhibit relatively high solubility (see Figure 5). In this case, the solubility of the solvent mixtures (see Figure 5) was generally better than that of the single-solvent systems (see Figure 3).
Form II was not observed at all in any solvent. The endotherm at 203 °C was the melting point of Form I, which might have been produced by the enantiotropic transformation of Forms II, III, IV (and possibly V) upon heating in the DSC (27). For example, a specimen of pure Form III generated in water that was free from seeds of Form I surpassed the transition point, melted at its melting point, and immediately underwent exothermic recrystallization, as indicated by the exotherm at about 182 °C in Figure 8k. But sometimes crystals did not resolidify as polymorphs with higher melting points when the system was kinetically trapped, as in the cases of sulfathiazole solids grown in THF and DMF (see Figures 8b and 8g) (28, 29).



Using the reported relative solubilities in the order of I > II > IV > III, the thermodynamic stability of the structures should increase in the order of I < II < IV < III (16). For sulfathiazole, crystallization from any solvent at a given temperature should initially give rise to the least stable form, followed by the stepwise conversion through the other metastable forms to the thermodynamically most stable Form III. But specific solvent–solute interactions might be needed to stabilize a particular form by inhibiting the nucleation and growth of the next most stable structure. Anwar reported that n-propanol could interfere with the completion of the β-sulfathiazole dimer in structures of Forms II, III, and IV because of its donor–acceptor duality (17). This could be why the efficiency of blocking the formation of a β ring in structures of Forms II, III, and IV increased in the order of water < methanol < ethanol < n-propanol < benzyl alcohol, as reflected by the decreasing amount of Forms III and IV in the DSC profiles (see Figures 8d, 8f, 8h, 8j, and 8k). On the other hand, solvents such as THF and DMF that lack a donor hydrogen and have lower Hansen hydrogen-bonding parameter (δh) values were unable to stabilize the transition state and gave a pure Form III structure without any trace of Form I seeds (see Figures 8b and 8g).
The kinetics effects of solvents on the crystallization of sulfathiazole polymorphs were also noticed in binary and ternary systems (16). Although sulfathiazole forms solvates, TGA scans verified that all sulfathiazole crystals produced were neither solvates nor hydrates because no weight loss upon heating was observed before the melting point of 203 °C (19). DSC responses of the sulfathiazole crystals grown in the 13 solvent mixtures of (acetonitrile:n-propanol:water) in Figure 9 showed that the crystals contained (polymorphs): (100:0:0) (Forms I, III, and IV), (0:100:0) (Forms I and V), (0:0:100) (Forms I and III), (50:50:0) (Forms I, III, and IV), (80:10:10) (Forms II, III, IV, and V), (45:45:10) (Forms I and V), (10:80:10) (Forms I, III, and IV), (60:20:20) (Forms I and V), (20:60:20) (Forms I, III, and IV), (33:33:33) (Forms II and III), (10:45:45) (Forms I and VI), (0:50:50) (Forms I, III, and IV), (45:10:45) (Forms I, III, and IV), (20:20:60) (Forms I, III, and IV), (50:0:50) (Forms I, III, IV, and V) and (10:10:80) (Forms I, III, and IV).
The endotherm at 203 °C was the melting point of Form I, which also might have been produced by the enantiotropic transformation of Forms III, IV, V, and VI upon heating in the DSC (29). But this time, Form II was observed for solids generated by the ternary solvent mixtures of (80:10:10) and (33:33:33) (see Figures 9e and 9j). Moreover, an endotherm that appeared at a relatively low 135 °C for the ternary solvent mixture of (10:45:45) (see Figure 9k) was considered a newly discovered polymorph for sulfathiazole, conventionally Form VI (29).
The crystal habits and length–breadth aspect ratios of sulfathiazole crystals produced by the 11 pure single solvents and 13 solvent mixtures of acetonitrile, n-propanol, and water by cooling are shown in Figures 10 and 11, respectively. In general, Form I possessed a needle-like morphology. Form II had a cuboid morphology. The morphology of Form III was a truncated hexagon and Form IV had a plate-like hexagonal morphology (9). If crystal habits alone are used as a visual guide, the major phases grown from the 11 pure single solvents were: Form I in MEK, acetone, benzyl alcohol, acetonitrile, and n-propanol; Form III in THF, DMF, ethanol, DMSO, and water; and Form IV in methanol. Clearly, the observation that Form I appeared with the same crystal habit from more than one solvent suggested that none of the available solvent–surface interactions could inhibit the addition of sulfathiazole molecules to the {010} face (9). A similar conclusion could be drawn for Form III and its fast-growing {110} face (9).
In the solvent mixture systems of acetonitrile:n-propanol:water, Form I was the major phase in the needle-like crystals grown in the solvent mixtures of (50:50:0), (45:45:10), (60:20:20), (0:50:50), and (50:0:50), judging by the crystal habits. Form III was the major phase in the truncated hexagonal crystals produced by mixtures of (80:10:10), (10:80:10), (33:33:33), (20:20:60), and (10:10:80). Form IV was the major phase in the plate-like hexagonal crystals generated in the solvent mixtures of (10:80:10), (10:45:45), (45:10:45), and (10:10:80). Unlike the crystal habits in the pure solvent systems, not all of the crystal habits in the solvent mixtures were well defined.The authors speculated that the lack of definition was caused by the presence of various polymorphs in a crystal, as indicated by the DSC scans (see Figures 8 and 9), and not by the solvent–surface interactions. The average size of crystals grown in the solvent mixtures was larger than those grown in pure solvent systems. Because the DSC traces in Figures 8 and 9 were derived from the sulfathiazole crystals produced by spontaneous nucleation, if the high crystal yield given by a solvent mixture is desired, the polymorphic purity of sulfathiazole can be ensured by seeding (3).ConclusionSuccessful large-scale preparation of fine chemicals, and the manufacture of pharmaceuticals in particular, depends heavily on the solubility, crystal yield, and polymorphism of solid compounds and active pharmaceutical ingredients. The cocktail solvent screening method is a simple and inexpensive technique on a laboratory scale. It should be implemented together with the initial solvent screening approach to enhance the solubility of green solvents, increase crystal yield, and optimize the chances of finding the number of polymorphs by coupling with DSC. |



References
1. C. Sanchez, H. Arribart, and M.M. Giraud-Guille, “Biomimetism and Bioinspiration as Tools for the Design of Innovative Materials and Systems,” Nature Mater. 4 (4), 277–288 (2005).
2. J. W. Schroer and K.M. Ng, “Process Paths of Kinetically Controlled Crystallization: Enantiomers and Polymorphs,” Ind. Eng. Chem. Res. 42 (10), 2230–2244 (2003).
3. W. Beckmann, “Seeding the Desired Polymorph: Background, Possibilities, Limitations, and Case Studies,” Org. Proc. Res. Dev. 4 (5), 372–383 (2000).
4. R. A. Granberg and Å.C. Rasmuson, “Solubility of Paracetamol in Pure Solvents,” J. Chem. Eng. Data 44 (6), 1391–1395 (1999).
5. R. A. Granberg and Å.C. Rasmuson, “Solubility of Paracetamol in Binary and Ternary Mixtures of Water + Acetone + Toluene,” J. Chem. Eng. Data 45 (3), 478–483 (2000).
6. D.J.C. Constable, C. Jimenez-Gonzalez, and R.K. Henderson, “Perspective on Solvent Use in the Pharmaceutical Industry,” Org. Proc. Res. Dev. 11 (1), 133–137 (2007).
7. J.W. Mullin, Crystallization, (Butterworth Heinemann, Boston, MA, 3rd ed., 1997), pp. 90–92.
8. J. Breu et al., “Nucleation-Controlled Crystallization of a New, Spontaneously Resolved Solvate of [Ru(bpy)3](PF6)2 and its Desolvation Reaction,” Chem. Eur. J. 8(19), 4454–4460 (2002).
9. N. Blagden et al., “Crystal Chemistry and Solvent Effects in Polymorphic Systems Sulfathiazole,” J. Chem. Soc. Faraday Trans. 94 (8), 1035–1044 (1998).
10. A. Kordikowski, T. Shekunov, and P. York, “Polymorph Control of Sulfathaizole in Supercritical CO2,” Pharm. Res. 18 (5), 682–688 (2001).
11. T. Lee and M.S. Lin, “Sublimation Point Depression of Tris(8-hydroxyquinoline) aluminum (III) (Alq3) by Crystal Engineering,” Cryst. Growth Des. 7 (9), 1803–1810 (2007).
12. Y. Kawashima, M. Okumura and H. Takenaka, “Spherical Crystallization: Direct Spherical Agglomeration of Salicylic Acid Crystals During Crystallization,” Science 216(4550), 1127–1128 (1982).
13. R.A. Granberg and Å.C. Rasmuson, “Crystal Growth Rates of Paracetamol in Mixtures of Water + Acetone + Toluene,” AIChE Journal 51 (9), 2441–2456 (2005).
14. M.L. Peterson et al., “Iterative High-Throughput Polymorphism Studies on Acetaminophen and an Experimentally Derived Structure for Form III,” J. Am. Chem. Soc. 124 (37), 10958–10959 (2002).
15. J.E. Anderson et al., “Determination of the Onset of Crystallization of N1-2-(thiazolyl)sulfanilamide (sulfathiazole) by UV–Vis and Calorimetry Using an Automated Reaction Platform; Subsequent Characterization of Polymorphic Forms Using Dispersive Raman Spectroscopy,” Spectrochimica Acta Part A 57 (9), 1793–1808 (2001).
16. S. Khoshkhoo and J. Anwar, “Crystallization of Polymorphs: the Effect of Solvent,” J. Phys. D: Appl. Phys. 26 (8B), B90–B93 (1993).
17. J. Anwar, S.E. Tarling, and P. Barnes, “Polymorphism of Sulfathiazole,” J. Pharm Sci. 78 (4), 337–342 (1989).
18. D.C. Grove and G. Keenan, “The Dimorphism of Sulfathiazole,” J. Am. Chem. Soc.63 (1), 97–99 (1941).
19. A.L. Bingham et al., “Over One Hundred Solvates of Sulfathiazole,” Chem. Commun. (7), 603–604 (2001).
20. E. Tedesco, D. Giron, and S. Pfeffer, “Crystal Structure Elucidation and Morphology Study of Pharmaceuticals in Development,” Cryst. Eng. Comm. 4 (67), 393–400 (2002).
21. N. Blagden and R.J. Davey, “Review: Polymorph Selection—Challenges for the Future?” Cryst. Growth Des. 3 (6), 873–885 (2003).
22. D. Winn and M.F. Doherty, “Modeling Crystal Shapes of Organic Materials Grown from Solution,” AIChE J. 46 (7), 1348–1367 (2000).
23. S. Byrn, K. Morris, and S. Comella, “Reducing Time to Market with a Science-Based Product,” Pharm. Technol. Outsourcing Resources for the Pharmaceutical Industry supplement, 46–56 (2005).
24. T. Lee, C.S. Kuo, and Y.H. Chen, “Solubility, Polymorphism, Crystallinity, and Crystal Acetaminophen and Ibuprofen by Initial Solvent Screening,” Pharm. Technol. 30(10), 72–92 (2006).
25. N.G. Anderson, Practical Process Research and Development , (Academic Press, New York, 2000), pp. 81–111.
26. E.B. Hershberg and J.E. Christensen, “Manufacture of Soluble Sulfathiazole,” US Patent 4070356, (1978).
27. A. Burger and R.D. Dialer, “Neue Untersuchungsergebnisse zur Polymorphic von Sulfathiazol,” Pharm. Acta Helv. 58 (3), 72–78 (1983).
28. T.L. Threlfall, “Analysis of Organic Polymorphs: A Review,” Analyst 120 (10), 2435–2460 (1995).
29. D. Giron, “Thermal Analysis and Calorimetric Methods in the Characterization of Polymorphs and Solvates,” Therm. Acta 248, 1–59 (1995). |
Advancing Approaches in Detecting Polymorphism
Identifying polymorphs is a crucial part of the drug-development process as researchers forward select methods to improve detection.
Screening and detecting polymorphism when developing and manufacturing active pharmaceutical ingredients (APIs) is an ongoing challenge for pharmaceutical manufacturers. Polymorphism is the ability of a compound to exist in more than one crystalline structure. Different solid forms can possess different properties, including solubility, which can in turn affect the bioavailability of the drug.
Polymorph screening and detection
“The investigation of polymorphic forms of a drug substance is valuable to the pharmaceutical industry, as the differences in the physical properties of different solid forms can be problematic in the later stages of development and formulation,” says Chris Frampton, chief scientific officer at SAFC-Pharmorphix (Cambridge, UK). “Different solid forms can show differences in properties such as aqueous thermodynamic solubility, melting point and hygroscopicity, which in turn can affect bioavailability, processability, and stability, respectively.”
One of the more well-chronicled examples of polymorphism occurred in ritonavir, the API in “Norvir,” a protease inhibitor developed by A bbott Laboratories (Abbott Park, IL). The drug was approved in 1996, and in mid-1998, Abbott encountered manufacturing difficulties with the capsule formulation, according to the company’s 1998 annual report. Ritonavir exhibited conformational polymorphism of two unique crystal lattices that had significantly different solubility properties (1). The formation of the polymorph caused Abbott to pull the drug from the market and reformulate.A recent analysis by SSCI, the solid-state chemistry business of Aptuit (Greenwich, CT), showed that of 245 compounds it has screened, 89% had multiple solid forms (see Figure 1). Approximately 50% of the compounds showed polymorphism, 37% were hydrates, and 31 were solvates, outlines Stephen Byrn, head of the scientific advisory board of Aptuit and head of the Department of Industrial and Physical Pharmacy at Purdue University.Other research conducted by Professor Ulrich Griesser at the University of Innsbruck shows a lower incidence of multiple solid forms in organic molecules: polymorphism (36%), hydrates (28%), and solvates (10%) (2).
“The difference in prevalence of 51% versus 36% probably reflects the fact that many organic chemists do not search for polymorphs when preparing new compounds,” explains Byrn. “In contrast, compounds submitted to SSCI/Aptuit are drug candidates. Another factor that may explain the differences in prevalence of polymorphs is the fact that SSCI/Aptuit would tend to get problem compounds, including materials that have a large numbers of polymorphs.”

Challenges in polymorph screening
A review of several APIs and new chemical entities (NCEs) shows not only the prevalence of other solid forms but also the challenges in detecting these forms.
 Figure 2 |
Acetaminophen. The polymorphism of acetaminophen shows the challenges in screening for polymorphs (see Figure 2).“The crystal structures for two known crystal forms of acetaminophen (monoclinic and orthorhombic) have been published,” explains Frampton (3, 4). “The monoclinic form is the thermodynamically stable crystal form at room temperature with respect to the orthorhombic form. Evidence for a third, metastable, form has been published (5, 6). To date, however, it has not been possible to isolate any crystalline material to enable a full crystal structure or physicochemical property determination.” |
“It is clear that in this case, conventional solvent-screening techniques of the time would fail to produce any evidence of the less-stable orthorhombic form since it was only possible to produce seeds of the orthorhombic form under somewhat extreme conditions,” says Frampton. “Given that the crystal structures of the two modifications were available, it was reasonably straightforward to identify the pure forms from their X-ray powder diffraction (XRPD) patterns (see Figures 3a and 3b). The form assignment from XRPD was subsequently confirmed by single-crystal X-ray analysis.The problem with acetaminophen was the repeated failures of research groups to isolate the orthorhombic form using the method of slow evaporation from ethanol as described by Haisaet al. (3), explains Frampton. Eventually, it was discovered that the orthorhombic form could be produced from solution by using seeds of the orthorhombic modification that were isolated from melt-crystallized acetaminophen (7).

 Figure 4 |
Sodium diclofenac. Sodium diclofenac (see Figure 4) is a nonsteroidal anti-inflammatory drug that shows polymorphism. The sodium salt of the drug is manufactured by Novartis as “Voltaren.” Frampton says there are three known anhydrous crystal modifications of the free base: two monoclinic forms (C2/c, P21/c), and an orthorhombic form (Pcan) (8–10).“Sodium diclofenac has also been shown to readily form stable hydrate phases when crystallized from aqueous miscible solvents,” explains Frampton (11). “Although these hydrated materials are not technically polymorphic phases since they are solvates, they do present interesting solid-form challenges.”


“We were prompted to reinterpret the single-crystal X-ray data after repeated thermogravimetric analysis (TGA) and Karl Fischer (KF) measurements indicated that the water content was reproducibly always slightly less (e.g., 21.48% and 21.0% from TGA and KF, respectively) than the theoretical content of 22.07% based a fully hydrated pentahydrate structure,” says Frampton. These values equate to 4.83 and 4.69 moles of water respectively. Figure 5 shows an example TGA.
“Redetermination of the single-crystal X-ray structure reveals that the structure contains only a pseudomirror plane, and as such, the true space group of the structure is P21and not P21/m. The asymmetric unit of the structure now contains four molecules of sodium diclofenac and 19 molecules of water, yielding an overall stoichiometry of a 4.75 hydrate,” says Frampton.
All the atoms in the crystalline asymmetric unit are now fully ordered, and all hydrogen atoms, including those on the 19 water molecules, were located in the Fourier difference maps and were included within the refinement. The theoretical water content for a fully hydrated 4.75 hydrate is 21.19%, which is in accord with the other analytical data, Frampton explains. The structure now has a much-improved crystallographic Rfactor of 3.04%.
“It is important to stress in this case that the correct result was only obtained after the consideration of all the analytical data and that we should not be surprised by the noninteger value of the water content,” he says. A further hydrated species, a triclinic 3.50 hydrate, was also identified in the study.
Andolast. Andolast, an NCE being developed by Rottapharm S.P.A. (Monza, Italy) for certain respiratory disorders, shows the challenge in polymorph screening and the potential opportunities for discovering and patenting a polymorph.
Andolast is administered as a dry powder for inhalation. “When a product is used in the form of micronized powder for the treatment of respiratory tract disease, the particle-size distribution (PSD) of the inhaled drug is crucial to its performance,” explains Markus von Raumer, product manager of the solid-state development unit at Solvias AG (Basel, Switzerland). “Physical chemical characteristics play a fundamental role in the case of inhaled drugs not only for the solubility issues shared with oral drugs but also due to the aggregation phenomena, which can impact negatively the performance of the powder, ultimately affecting its pharmacological activity,” he says (12).
During the early pharmaceutical development of andolast, deviations in the consistency of the API’s physical chemical characteristics were observed, and a review of the solid-state issues was required, explains von Raumer. These deviations related to: batch-to-batch PSD variability (which affected released fraction and therefore drug efficacy); batch-to-batch variability in hygroscopicity (which affected released fraction and hence manufacturability); and batch-to-batch variability in chemical–physical characteristics that reflected in flow and blend properties (which affected manufacturability and therefore product cost).
Analysis of the complete batch history, combined with fresh physicochemical analysis of key samples, revealed several issues. Deviations occurred independently upon the supplier, upon the batch scale, and upon the batch purity. For all batches, the same chemistry and the same isolation procedure was used.
“Sequential to compilation of historic data, the crystallization conditions and a crystallization process investigation was carried out,” says von Raumer. The screening was based upon product precipitation from several water-miscible solvents changing concentration, water content, and temperature. Phase-equilibration experiments were carried out as well. In these experiments, material from the previous batches was slurried in different solvents, at different water concentrations, different temperatures, and for different times. Crystallization from different media gave different solids, in terms of XRPD, Fourier transform (FT)-Raman spectra, and hygroscopicity.
Crystallization in thermodynamic conditions, however, afforded the same product when a proper amount of water was present, points out von Raumer. This product was not hygroscopic, was stable, and its water content determined by KF was about 20%. Crystallization under kinetic conditions, especially when the water activity in the medium was poor, afforded a hygroscopic product with KF lower than 20%. This product was not stable and converted under appropriate conditions into a stable product with KF of approximately 20% and XRPD and Raman results identical with the precipitated product. All the products obtained from crystallization trials that gave KF around 20% displayed the same XRPD spectrum.
“According to the above experiments, it was proven that andolast disodium crystallized in only one preferred hydrated form: the penthaydrate. All other hydrated forms were not stable and converted under appropriate conditions into the pentahydrate,” notes von Raumer. “The single-crystal structure was resolved, and molecular-mechanics simulation showed that, upon water removal, a certain degree of distortion was introduced within the crystal structure, with the distortion being proportional to the number of water molecules removed.”
From this solid-state study, a new crystalline form, andolast pentahydrate, was revealed, which displayed certain superior properties, says von Raumer. Andolast pentahydrate is not a hygroscopic solid, is stable to mechanical milling, and is stable to as much as 85% relative humidity. It also can consistently be prepared according to a standard process, has constant mechanical properties such as flowability, and has advantages in formulation in comparison with previous material because of a better performance in delivered dose, fine particles delivered, and fine-particle fraction. Aside from improved performance, the new crystalline form of andolast also led to patent applications in the United States and Europe (13, 14). The European patent application calls for an expiration date of 2026 compared with the original expiration date of 2010 that is specified in an earlier patent (15).
“While the development of the andolast drug product was hampered due to inconsistencies and was slowed down by a non-negligible factor, the actual situation allows for a rapid continuation with a possibly much longer time horizon of exclusivity,” says von Raumer. “This situation once again demonstrates the utility and opportunities that are created by carefully investigating the solid-state aspects of drug candidates.”
Analytical methods for polymorphs
XRPD is the most common analytical method used in polymorph screening, although certain researchers use Raman spectroscopy as their primary screening method. “Each method has its value, and more recently SAFC-Pharmorphix has been experimenting on the use of both methods in combination for polymorph and cocrystal screening using a combined X-ray powder diffractometer with a Raman attachment,” says Frampton. “This instrument has been developed in collaboration with Bruker AXS, and a patent covering this technology has recently been published (16). This technology has been further enhanced by a new release of the clustering program, Polysnap2, which can cluster any two-dimensional data and produce weighted combinations.” SAFC-Pharmorphix has an active collaboration with Professor Chris Gilmore and his group at the University of Glasgow (Glasgow, UK).
In a typical study, a crystallographer places a compound in a range of solvents and subjects them to a range of crystallization conditions in hopes of obtaining single crystals, Aptuit’s Byrn explains. He points out that the number of solvents used in the screening varies. A small polymorph screen should include 8–10 solvents (17). “A more complete screen would require more than 50 solvents,” he adds. In recent research, Xu and Redman-Furey outlined an approach to narrow their selection of 57 solvents to 20 (18). Byrn points out that in a related study, Miller suggested an approach of finding the most stable polymorphs by slurring compounds in a variety of solvents (19).
“We now know that solvent-based crystallizations alone do not find all of the forms. In several cases, solvent-based polymorph screens have missed very important solid forms,” explains Byrn. In another example, Yu discovered the second most stable form of 5-methyl-2-[(2-nitrophenyl)amino]-3-thiophenecarbonitrile (ROY) using a melt-recrystallization technique (20). “It is well established that ROY had previously been screened by all of the best-known high-throughput techniques, and this form had not been found,” explains Byrn, adding, solvent-free techniques such as drying, sublimation, and amorphous crystallization can produce new forms not discovered by solvent-based crystallization.
Byrn offers several examples of techniques used to find polymorphs. In a study at Aptuit/SSCI, nabumetone was recrystallized from a wide range of solvents under different conditions. About 250 experiments were performed, yielding Form I. When nabumetone was recrystallized in capillaries, a procedure known to produce very high supersaturations, a new form was discovered in about 70 of 400 crystallizations. Analysis of these crystallization conditions shows that the appearance of the new form depends on superaturation and quiescence and not on solvent content, Bryn explains.
In another study, SSCI/Aptuit compared the polymorphs formed in a common high-throughput technology with those from capillaries and traditional crystallizations under a broad range of conditions. “It was clear that the plate crystallization technology, which is commonly used in high-throughput polymorph screens, tends to overproduce one form relative to other methods,” says Byrn.
Other methods at work
Although XRPD is the most common technique used in the overall solid-state characterization of pharmaceutical materials, other techniques are needed to understand the form, determine how it behaves under stress conditions, discern the relationship between forms, and decide which form is suitable for development.
“The powder pattern will tell you if it is crystalline but will not provide critical information such as solvation state, melting point, water uptake, solubility, and physical stability, for example,” explains Byrn. “Thermal data such as differential scanning calorimetry, thermogravimetry, and hot-stage microscopy are used to determine melting temperature, solvation state, desolvation, and form changes upon drying. This information can be directly related to processing such as drying.”
Gravimetric vapor sorption is used to measure water sorption and desorption, which can lead to environmental handling guidelines to prevent hydrate formation or dehydration upon exposure to various relative humidity conditions. Other methods such as infrared, Raman, and nuclear magnetic resonance (NMR) spectroscopies can often show specificity between forms that may be more difficult to see with XRPD.
“These techniques, along with XRPD and others, are commonly used for quantitative solid-state method development to quantitate the amount of different forms present in API or drug product or confirm that only one form is present. It is usually desired to have only one form produced, but processing such as drying, milling, and granulation, can commonly lead to multiple forms, indicating that an understanding of form changes during the process and better control of the process is needed,”explains Byrn.
He points to recent advances in screening methodology using Raman microscopy to determine the presence of multiple forms in the same crystallization vessel. “Additionally, NIR is finding some use as a tool for differentiating polymorphs during screening. Finally, the developments of X-ray microscopy at Argonne National Laboratories [Argonne, IL] and other synchrotron sources promises to increase our ability to detect solid forms in small samples.”
Other spectroscopic methods may be used in detecting polymorphs. “Like infrared spectroscopy, where the absorption of infrared energy is characteristic of a particular molecular vibration, the absorption of the radio-frequency radiation is characteristic of the magnetic environment of the nuclei, explains SAFC-Pharmorphix’s Frampton. When applied to solids, cross-polarization magic-angle spinning solid-state nuclear magnetic resonance spectroscopy (CPMAS SS NMR) can give useful information regarding the crystal structure and polymorphic form of the compound. It also can also reveal the degree of crystallinity of the sample under investigation, he says. “Crystalline samples yield narrow lines as equivalent nuclei are in a constant magnetic environment dictated by the crystal structure, whereas amorphous solids usually yield broader lines reflecting the range of environments found in the solid. CPMAS SS NMR spectroscopy can also give valuable information on the crystalline asymmetric unit of the crystal structure.”
References
1. J. Bauer et al., “Ritonavir: An Extraordinary Example of Conformational Polymorphism,” Pharm. Res. 18 (6), 859–866 (2001).
2. U. Griesser, “Relevance and Analysis of Polymorphism in Drug Development,” presented at British Association of Crystal Growth Spring Meeting. Lancaster, UK, Apr. 4–6, 2006.
3. M. Haisa, S. Kashino, and H. Maeda, “The Orthorhombic Form of p-Hydroxyacetanilide,” Acta Cryst. B30, 2510–2512 (1974).
4. M. Haisa et al., “The Monoclinic Form of p-Hydroxyacetanilide,” Acta Cryst. B32, 1283–1285 (1976).
5. A. Burger in Acta Pharm. Technol. 28 (1), 1–20 (1982).
6. P. DiMartino et al., ” Preparation and Physical Characterization of Forms II and III of Paracetamol,” J. Therm. Anal. 48 (3), 447–458 (1997).
7. G. Nichols and C.S. Frampton, “Physicochemical Characterization of the Orthorhombic Polymorph of Paracetamol Crystallized from Solution,” J. Pharm. Sci. 87(6), 684–693 (1998).
8. D Kovala-Demertzi, D. Mentzafos, and A. Terzis, “Metal Complexes of the Anti-inflammatory Drug Sodium [2-[(2,6-dichlorophenyl)amino]phenyl]acetate (diclofenac sodium,” Polyhedron 12 (11), 1361–1370 (1993).
9. C. Castellari and S. Ottani, “Two Monoclinic Forms of Diclofenac Acid,” Acta Cryst.C53, 794–797 (1997).
10. N. Jaiboon et al., “New Orthorhombic Form of 2-[(2,6-dichlorophenyl)amino]benzeneacetic acid (Diclofenac Acid),” Anal. Sci. 17, 1465–1466 (2001).
11. N. Muangsin et al., “Crystal Structure of a Unique Sodium Distorted Linkage in Diclofenac Sodium Pentahydrate,” Anal. Sci. 18, 967–968 (2002).
12. P.J. Akins, “Dry Powder Inhalers: An Overview,” Resp. Care 50 (10), 1314–1312 (2005).
13. A. Giordani et al., “New Crystalline and Stable form of Andolast,” US Patent Application 20070149586, June 28, 2007.
14. A. Giordani et al.,”A New Crystalline and Stable Form of Andolast,” EP Application 06112427.7, Apr. 10, 2006.
15. F. Makovec et al., “Derivatives of N-Phenylbenzamide with Anti-Ulcer and Anti-Allergy Activity and a Method for their Preparation, ” WO 9009989 PCT, Sept. 7, 1990.
16. C.S. Frampton et al., “Combinatorial Screening System with X-ray Diffraction and Raman Spectroscopy,” US Patent US 2006/0023837 A1, Feb. 2, 2006.
17. S.R. Byrn, “Pharmaceutical Solids: A Strategic Approach to Regulatory Considerations,” Pharm. Res. 12, 945–954 (1995).
18. D. Xu and N. Redman-Furey, “Statistical Cluster Analysis of Pharmaceutical Solvents,” Intl. J. of Pharm. 339 (1-2), 175–188 (2007).
19. J.M. Miller et al., “Identifying the Stable Polymorph Early in the Drug Discovery-Development Process,” Pharm. Dev. Technol. 10 (2), 291–297 (2005).
20. S. Chen, I.A. Guzei, and L. Yu, “New Polymorphs of ROY and New Record for Coexisting Polymorphs of Solved Structures,” J. Am. Chem. Soc. 127 (27), 9881–9885 (2005).
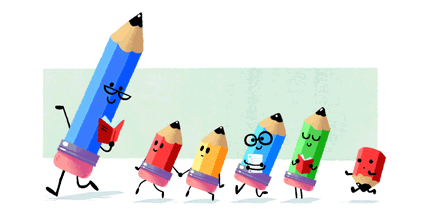
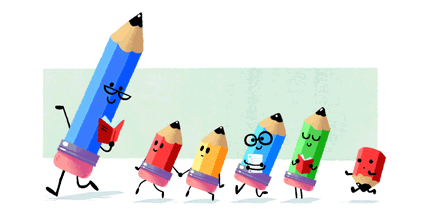
DR ANTHONY CRASTO, PhD, ICT Organic chemistry, Currently working with GLENMARK GENERICS LTD research centre as Principal Scientist, process research (bulk actives) at Mahape, Navi Mumbai, India, helping millions, 9 million hits on google on all organic chemistry websites, Hands on experience in developing novel routes for drug molecules and implementation on commercial scale. several international patents published.pushing boundaries, 2.5 lakh connections on all networking sites

MOBILE-+91 9323115463
GLENMARK SCIENTIST , NAVIMUMBAI, INDIA
235,192 total views, 12 views today